Introduction
Soil acidity (pH < 5.5) significantly limits plant growth and yield. Approximately 30% of the world’s land area comprises acidic soils, which are found in both hemispheres (Von Uexküll and Mutert 1995). Low soil pH considerably impacts the availability of nutrients and other soil elements for plant uptake. A primary effect of low pH is the dissociation and release of the soluble Al ion (Al 3+), which is readily absorbed by plants, causing toxicity (Kochian et al. 2005).
The root is the first organ contacting the toxic Al 3+. Rhizotoxic Al 3+ concentrations inhibit cell division in the tips of main and lateral roots, increase cell wall rigidity by cross-linking pectins, and inhibit root elongation (Kopittke et al. 2016). These effects interfere with water and nutrient uptake. Consequently, the degree to which rhizotoxic concentrations of Al 3+ suppress root growth is a primary determinant of the plant’s overall response to Al. Staining intensity of root tips with hematoxylin, a dye that localizes Al, correlates with the Al-sensitivity of plants (Barceló and Poschenrieder 2002) and is used to rank species and genotypes according to Al susceptibility (Cançado et al. 1999).
Plants growing in acidic soils have evolved mechanisms to withstand low pH and Al toxicity (Poschenrieder et al. 2008, Chandra and Keshavkant 2021). Among these plants, certain species have a high capacity to accumulate Al in their leaves, i.e., “Al accumulators”. The Al concentration in the aerial parts of accumulator species exceeds the critical value of 1 mg g –1DW and the plants exhibit efficient internal detoxification mechanisms, including Al 3+ chelation with low molecular weight compounds, especially organic acids and phenolic compounds (Poschenrieder et al. 2015). Al accumulator species have primarily been found in the acidic soils of the humid tropics, which are covered by savannahs and tropical rain forests. These species are predominantly shrubs and trees of Melastomataceae, Polygonaceae, Theaceae, Caesalpinaceae, Rubiaceae, and Hydrangeaceae (Jansen et al. 2002, Poschenrieder et al. 2015). Currently, there is limited knowledge regarding the status of Al accumulation in the flora of acid soils from outside the tropics.
In Iran, tea cultivation is restricted to regions with acidic soils in the south of the Caspian Sea, north Iran. In these tea plantations, leaching through rainfall and the use of ammonium fertilizers have drastically decreased the soil pH to values between 4.0 and 4.5. Numerous plant species are growing as weeds in these tea gardens, favored by the moderate temperatures, high humidity, and abundant rainfall. However, there is no information on Al accumulation and tolerance in this weed flora.
Amaranthaceae is a prevalent cosmopolitan family found from the tropics to cool temperate regions. This botanical family consists of annual or perennial plants, primarily herbs, but also occasionally shrubs, small trees, and vines (Simpson 2010). After the combination of Amaranthaceae and Chenopodiaceae into a single family (Judd et al. 1999, Stevens 2001), the extended Amaranthaceae family comprises approximately 175 genera and 2,000 species and is considered the most species-diverse lineage within the Caryophyllales (Müller and Borsch 2005). Important economic crop plants, forage crops, fodder plants, vegetables, and euhalophytes can be found in the Amaranthaceae family, which thrives in various environmental conditions (Simpson 2010). Amaranthaceae contain approximately 50% of all known C 4 species among eudicots (Kadereit et al. 2003) and are distinguished by their diverse chemistry, which includes betalains, flavonoids, and phenolic acids (Mroczek 2015).
Amaranthus L. is a genus of about 70 annual monoecious and dioecious species with C 4 photosynthesis and global distribution (Kigel 1994, Kadereit et al. 2003). Several Amaranthus species are used as ornamentals ( A. caudatus, A. cruentus, A. hypochondriacus, A. tricolor), as medicinal plants ( A. blitum, A. caudatus, A. cruentus, A. dubius, A. hypochondriacus, A. spinosus, A. thunbergh, A. tricolor), vegetable leaves ( A. blitum, A. caudatus, A. cruentus, A. dubius, A. graecizans, A. hybridus, A. hypochondriacus, A. lividus, A. thunbergh, A. tricolor) or for food grains ( A. caudatus, A. cruentus, A. hypochondriacus) (Adegbola et al. 2020, Manyelo et al., 2020). Many are found as weeds in agricultural fields (Sellers et al. 2003). Despite their cosmopolitan behavior and the widespread human use, information is lacking on the adaptation to acid soils and Al accumulation capacity in the species of this genus, its family (Amaranthaceae), and order (Caryophyllales) (as per Jansen et al. 2002 and subsequently published works).
In northern Iran’s tea gardens, Amaranthus species are found as a weed (Hajiboland, unpublished data). The low pH and high Al 3+ availability in the soils of these tea plantations imply a high Al tolerance and likely Al-accumulating capacity. However, no studies have so far examined these features in these species. This study aimed to investigate Al accumulation and tolerance in four Amaranthus species to determine their soil acidity tolerance, Al toxicity, and Al accumulation capacity. Low and high Al treatment levels were applied in both short- and long-term experiments with a special emphasis on the root growth and morphology parameters as indicators of plant Al response. This study may extend our knowledge on Al tolerance and accumulation in Amaranthaceae, a family which has not yet been studied for Al response or accumulation.
Materials and methods
Collection of seeds and cultivation of plants
The seeds of A. blitoides and A. retroflexus were collected from acid soils of a tea garden (pH 4.5), N Iran, while the seeds of A. cruentus and A. tricolor were collected from calcareous soil, Khosrow-Shahr (pH 7.5), NW Iran.
The seeds were surface-sterilized with 10% (w/v) sodium hypochlorite and were germinated in perlite under dark conditions at 25 °C. Young seedlings were transferred to light and irrigated with 25% Hoagland nutrient solution (pH 6.0) (Johnson et al. 1957) for another week. Two-week old plants of similar sizes were transferred to aerated hydroponic pots filled with 100% Hoagland nutrient solution (pH 6.0) and precultured for two weeks.
Plants treatments
A short-term experiment was undertaken for evaluation of the effect of increasing Al concentrations on the shoot growth, various root growth parameters and some biochemical attributes in four Amaranthus species. Two-weeks after preculture, the nutrient solution was replaced with a low-strength nutrient solution (pH 4.0) containing macronutrients (mM): 1.2 KNO 3, 0.8 Ca(NO 3) 2×4H 2O, 0.1 NH 4H 2PO 4, 0.1 MgSO 4×7H 2O, 0.3 NH 4Cl, 0.1 MgCl 2 and micronutrients (µM): 10 KCl, 5 H 3BO 3, 0.4 MnSO 4×H 2O, 0.4 ZnSO 4×7H 2O, 0.1 CuSO 4×5H 2O, 0.1 H 2MoO 4, 4 Fe-EDTA with four Al levels (0, 20, 50, 200 and 400 µM as AlCl 3). The activity of free Al 3+ was 0, 3.75, 11.97, 60.34 and 125 µM respectively calculated by GEOCHEM-PC (Parker et al. 1995).
Three weeks after starting the treatments, plants were harvested and the fresh weight (FW) and dry weight (DW) of the shoots and roots were determined. The tap root length was measured with a ruler and the total length of roots was determined by the line intercept method (Tennant 1975). In addition, some physiological and biochemical parameters including leaf chlorophyll content, chlorophyll fluorescence, photosynthetic rate, anthocyanin concentration and activity of nitrate reductase were determined in the young leaves (the second and third youngest, fully-expanded leaves) of the experimental plants treated with 0, 50 and 400 µM Al as described below. Root staining was undertaken in plants treated with 0, 50, 200 and 400 µM Al according to the method described below.
A long-term experiment was undertaken for evaluation of the long-term effect of Al treatment on the biomass of and Al accumulation capacity in four Amaranthus species. Plants were grown with addition of 0, 50, 200 and 400 µM of AlCl 3 in the hydroponics as described above. Six weeks after start of the treatments, plants were harvested. In addition to measurement of the biomass, Al concentrations in the young (the second youngest) leaves and old (the second oldest) leaves were analyzed in the experimental plants as described below.
In both experiments, the plants were grown in a growth chamber with 16/8 h of light/dark photoperiod at 25/17 °C, relative humidity of 50–60%, and at a photon flux density of about 400 μmol m −2 s −1 provided by fluorescent lamps. The nutrient solutions were replaced weekly with fresh solutions.
Root staining
Haematoxylin staining of root apices was performed according to Polle et al. (1978). Roots were excised at 20-mm distance from the tip, rinsed with distilled water for 15 min, and subsequently placed in micro-tubes filled with 0.2% haematoxylin and 0.02% (w/v) potassium iodide (KI) for 20 min. Thereafter, the root tips were washed three times with distilled water, mounted on the glass slides and photographed.
Determination of leaf chlorophyll content, chlorophyll fluorescence and gas exchange parameters
The leaf chlorophyll content was measured as SPAD value with soil plant analysis development (SPAD) chlorophyll meter (SPAD-502, Minolta). The maximum quantum efficiency of PSII ( F v / F m ) was recorded in the attached leaves using a portable fluorometer (OSF1, ADC Bioscientific Ltd., UK). The net photosynthetic rate ( A) was determined using a calibrated portable gas exchange system (LCA-4, ADC Bioscientific Ltd., UK) during the light period between 9:00 and 13:00 h under a PPFD of approximately 300 μmol m –2 s –1 provided by incandescent lamps.
Determination of leaf anthocyanins
The leaf anthocyanin concentration was analyzed using a pH differential method (Tonutare et al. 2014). The leaves were extracted in methanol/HCl (98:2, v/v) and the homogenate was centrifuged at 5000 g. The supernatant was diluted (1:2) with two different buffers including 25 mM KCl (pH 1.0) and 400 mM Na acetate (pH 4.5). The absorbance of both solutions was determined at 510 and 700 nm and the anthocyanin content was expressed as the cyanidin-3-O-glucoside equivalents using its extinction coefficient of 26900 M –1 cm –1 (Tonutare et al. 2014).
Assay of nitrate reductase activity
Activity of NR (E.C. 1.6.6.1) was determined using the method described by Jaworski (1971). Leaf samples were cut into 5-10 mm sections and placed in incubation buffer (100 mg tissue for 10 mL of buffer) containing 25 mM potassium phosphate buffer (pH 7.2), 25 mM KNO 3 and 1% Triton X-100. The samples were infiltrated using a vacuum (80 kPa). After 3 h, the vacuum was released and the samples were incubated at 30 ºC in darkness for 1 h, then placed in a boiling water bath to stop the NR activity. The resulting nitrite was determined spectrophotometrically at 540 nm in a reaction mixture containing sulfanilamide and naphthylethylenediamine dihydrochloride (N-NEDA).
Elemental analyses
Oven-dried samples of the young and old leaves were ashed at 500 °C in a muffle furnace then were resolved in 10% HCl and made to volume by double-distilled water. The aluminum concentration was determined by an atomic absorption spectrophotometer (AA‐6300, Shimadzu, Japan).
Experimental design and statistical analyses
The experiment was undertaken using a completely randomized design with four independent pots as four replicates. Pairwise comparison of means was performed by Tukey’s test (P < 0.05).
Results
Plant biomass and root morphology after three weeks of growth in different Al concentrations
The shoot biomass of two species whose seeds were collected from acidic soils ( A. retroflexus and A. blitoides) was significantly improved with 20 and 50 µM Al, but was constricted under 200 and 400 µM Al (Fig. 1A, C). Like the shoot biomass, the root biomass was increased in plants exposed to 20 and 50 µM Al in both species. The highest Al concentration (400 µM) significantly decreased root biomass in both species, whereas 200 µM Al had no effect (Fig. 1B, D).
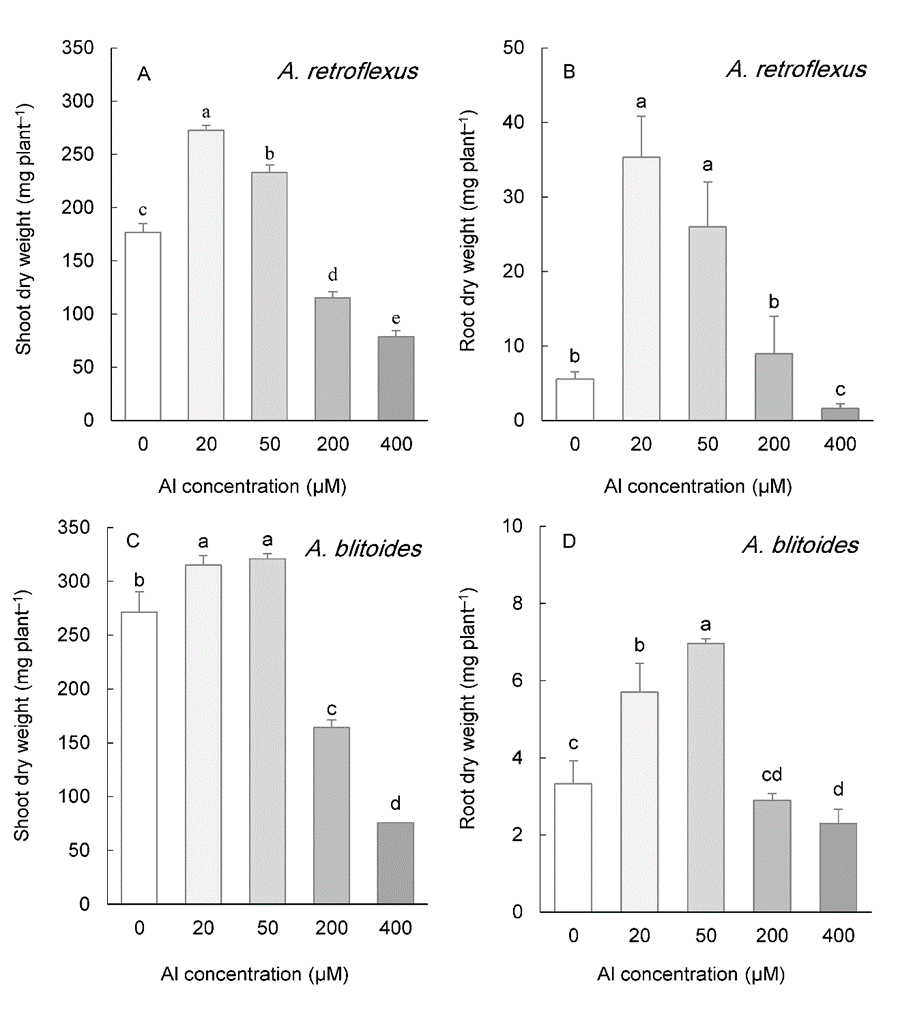
Fig. 1. Shoot and root biomass in Amaranthus retroflexus (A, B) and A. blitoides (C, D) grown for three weeks under different Al concentrations in the hydroponic medium. Data are mean values of 4 replicates ± standard deviation. Bars indicated by the same letters are not significantly different ( P < 0.05).
In the two species whose seeds were collected from calcareous soils ( A. cruentus and A. tricolor), low concentrations of Al (20 and 50 µM) also improved the shoot DW (Fig. 2A, C). This parameter was repressed by 400 µM Al in A. cruentus, but showed no effect in A. tricolor (Fig. 2A, C). Even at the highest Al concentration applied to the medium (400 µM Al), root biomass was not inhibited. The root DW increased at 50 µM Al in both species and at 200 µM Al in A. tricolor (Fig. 2B, D).
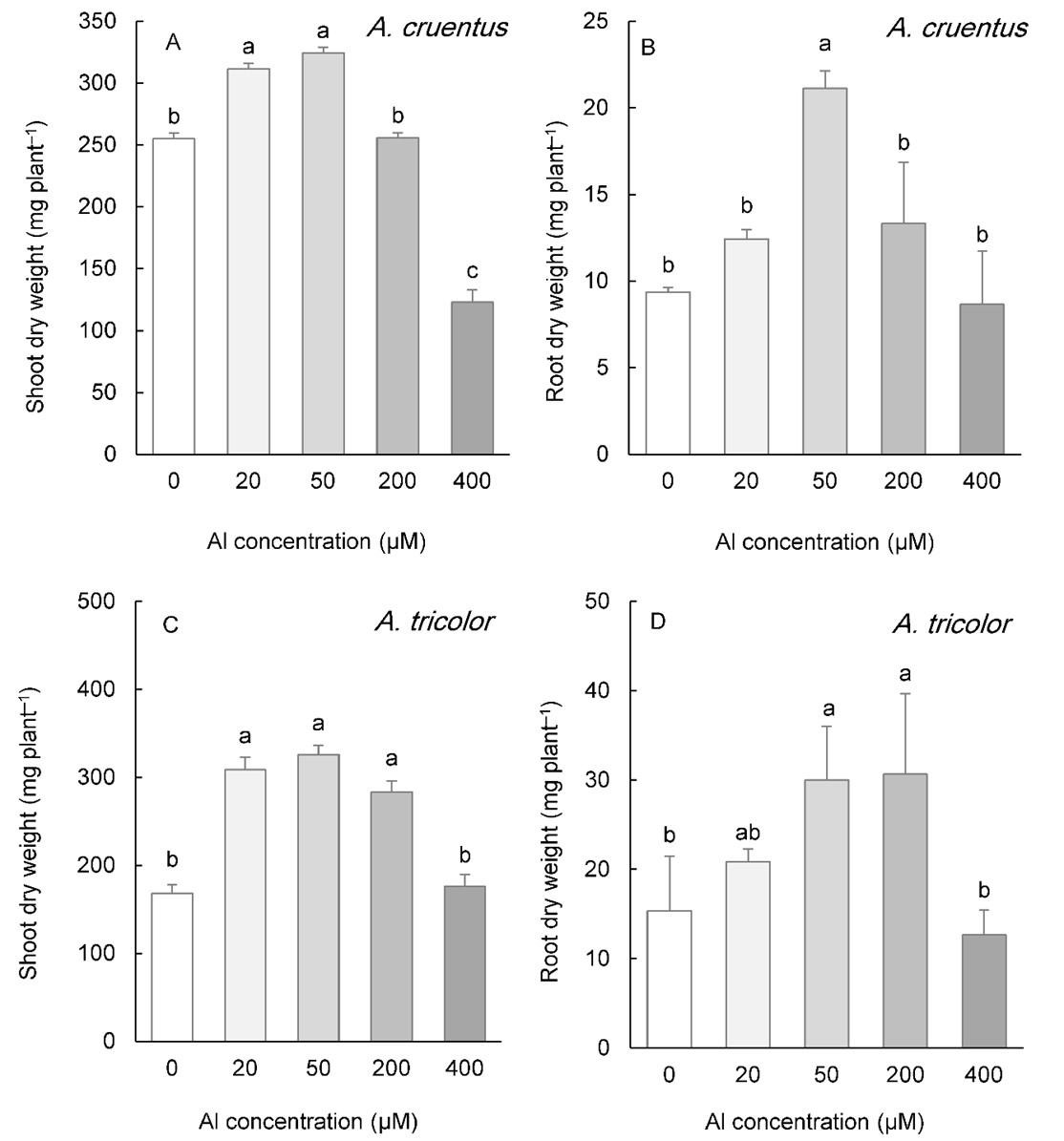
Fig. 2. Shoot and root biomass in Amaranthus cruentus (A, B) and A. tricolor (C, D) grown for three weeks under different Al concentrations in the hydroponic medium. Data are mean values of 4 replicates ± standard deviation. Bars indicated by the same letters are not significantly different ( P < 0.05).
Al treatment significantly influenced the root morphology of A. retroflexus and A. blitoides (Fig. 3). In A. retroflexus, both the taproot length and the total root length were stimulated by 20 to 200 µM Al, but inhibited by 400 µM Al. (Fig. 3A-C). The application of 20 µM Al to A. blitoides substantially increased the taproot length. However, total root length was increased with Al concentrations up to 50 µM. These parameters were not affected by 200 µM Al in this species but were significantly inhibited by 400 µM Al (Fig. 3D-F).
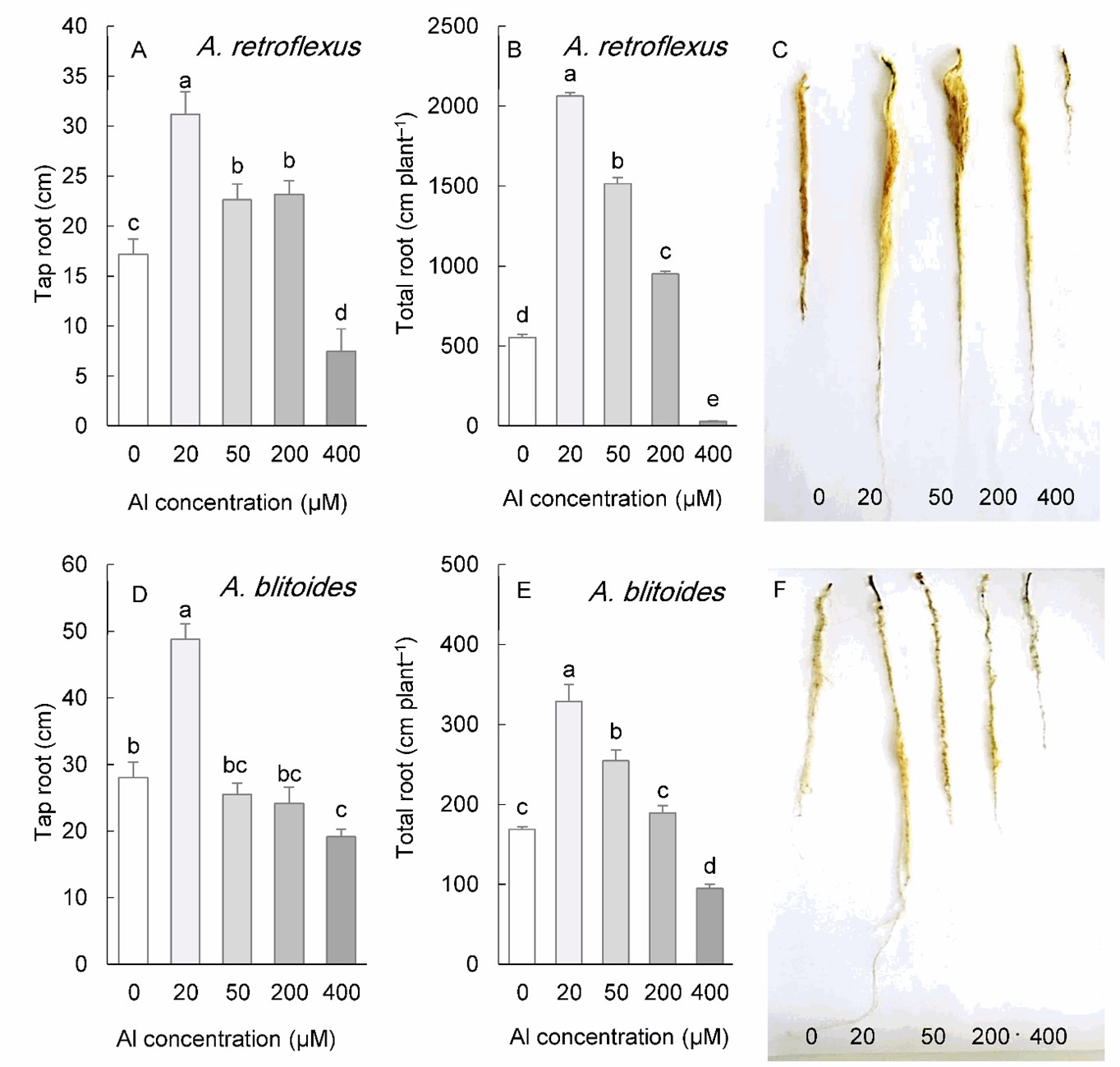
Fig. 3. Length of the tap roots and total length of the roots in Amaranthus retroflexus (A, B) and A. blitoides (D, E) grown for three weeks under different Al concentrations in the hydroponic medium. The right panels represent the roots of plants under different Al concentrations. Data are mean values of 4 replicates ± standard deviation. Bars indicated by the same letters are not significantly different ( P < 0.05).
A. cruentus and A. tricolor exhibited a similar effect of Al on root morphology (Fig. 4). Taproot length was significantly greater in plants exposed to 20 µM Al (in A. cruentus) or 20-50 µM Al (in A. tricolor), remained unchanged at 200 µM Al, but decreased significantly under 400 µM Al in both species (Fig. 4A, D). In response to a range of 20-200 µM Al concentrations, however, the total root length of both species was stimulated. Both species exposed to 400 µM Al exhibited a significant reduction in root length (Fig. 4B, E).
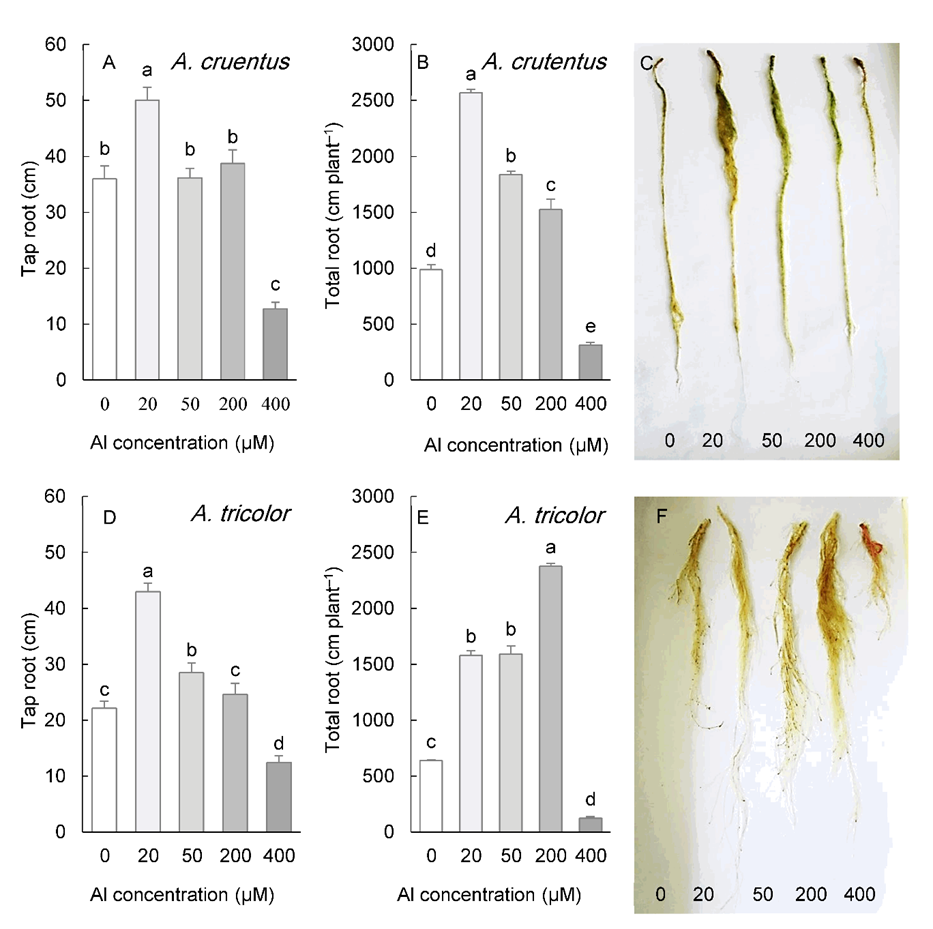
Fig. 4. Length of the tap roots and total length of the roots in Amaranthus cruentus (A, B) and A. tricolor (D, E) grown for three weeks under different Al concentrations in the hydroponic medium. The right panels represent the roots of plants under different Al concentrations. Data are mean values of 4 replicates ± standard deviation. Bars indicated by the same letters are not significantly different ( P < 0.05).
According to relative increase (% over control) in shoot growth under 50 µM Al, A. tricolor was the most responsive species with a 93% increase, followed by A. retroflexus with a 32% increase. A. retroflexus exhibited the greatest stimulation of root biomass (367%), total root length (173%), and taproot length (32%) (On-line Suppl. Fig. 1A). Al susceptibility based on the relative inhibition of shoot biomass by 400 µM Al followed the order A. blitoides > A. retroflexus > A. cruentus > A. tricolor (On-line Suppl. Fig. 2B). The inhibitory effect of Al on root growth was, however, dependent on the growth parameters, A. retroflexus was most sensitive to 400 µM Al as measured by root biomass and total root length, while it was the second most susceptible species in terms of taproot length. Similarly, A. cruentus was the most resistant to Al concerning root biomass, but the most sensitive for taproot length. In addition, the response of shoot growth was independent of that of root growth. A. blitoides was the most susceptible regarding the response of shoot biomass to 400 µM Al, whereas it was the most tolerant species regarding the response of taproot and total root length (On-line Suppl. Fig. 1B).
The principal component analysis (PCA) of growth parameters revealed a close relationship between the tap root length and total root length under low Al concentration (50 µM Al), while the root biomass was clustered separately. The shoot biomass response was also weakly correlated with root growth parameters under low Al concentration (On-line Suppl. Fig. 1C). Under higher Al concentration (400 µM), a poor correlation was observed between the shoot and root Al responses (On-line Suppl. Fig. 1D).
Root staining with hematoxylin following three weeks of growth at varying Al concentrations
The violet-blue color of the Al-haematoxylin complex was not observed in the roots of A. retroflexus plants treated with 50 µM Al. In A. blitoides and A. tricolor, only the 0-2 mm root tips were stained with hematoxylin, while in A. cruentus, both the root tip and basal parts (0-15 mm) were stained (On-line Suppl. Fig. 2). Except for A. cruentus, which displayed a uniform hematoxylin staining at 200 µM Al, the root tips of the other three species displayed a localized hematoxylin staining between 0 and 2 mm. Under the highest Al concentration applied to the plants (400 µM), the basal root parts of all four studied species stained visibly. However, the intensity of staining was significantly greater in A. blitoides and A. cruentus than in A. retroflexus and A. tricolor (On-line Suppl. Fig. 2).
Physiological and biochemical parameters after three weeks of growth at various Al concentrations
Low (50 µM) and high (400 µM) Al treatments decreased leaf SPAD values in A. retroflexus, whereas only high Al concentration affected this parameter in A. blitoides and A. cruentus. In A. tricolor, however, leaf SPAD values remained unaffected under either of the Al treatments (Tab. 1).
Tab. 1. Leaf chlorophyll content as SPAD (soil plant analysis development) value, maximum quantum yield of PSII ( F v / F m ), photosynthetic rate ( A, µmol CO 2 m –2 s –1), concentrations of anthocyanins (µg cyaniding-3-glucoside g –1 FW) and activity of nitrate reductase (NR, nmol NO 2 – mg –1 pr min –1) in the young leaves of four Amaranthus species grown hydroponically and treated with three different Al (0, 50 and 400 µM) concentrations for three weeks. Data of each column within each species indicated by the same letters are not significantly different ( P < 0.05).
The maximum quantum yield of PSII ( F v / F m ) decreased by the highest Al treatment (400 µM) in A. retroflexus and A. blitoides. This parameter was unaffected by Al levels in A. tricolor but increased in A. cruentus when a low Al concentration (50 µM) was applied (Tab. 1).
The photosynthesis rate was reduced by both Al concentrations (50 and 400 µM) in A. retroflexus and A. tricolor, it decreased at the highest Al level (400 µM) in A. blitoides, but was unaffected by any of the applied Al concentrations in A. cruentus (Tab. 1).
The concentrations of anthocyanins in the leaves of A. retroflexus were unaffected by Al, while a simulation was observed with increasing Al levels in the other three species. The constitutive concentrations of anthocyanin were highest in A. tricolor, followed by A. cruentus (Tab. 1) and lower in A. retroflexus and A. blitoides.
The leaf activity of NR increased under 50 µM Al in all four studied species (Tab. 1). The response of NR activity to higher Al concentration (400 µM) was species-dependent, it decreased in A. cruentus, remained unchanged in A. retroflexus and A. blitoides, and increased in A. tricolor (Tab. 1).
Biomass and Al accumulation following six weeks of growth at various Al concentrations
Under long-term cultivation (six weeks), Al concentrations below 400 µM did not significantly affect shoot growth in any Amaranthus species (Fig. 5 and Fig. 6).
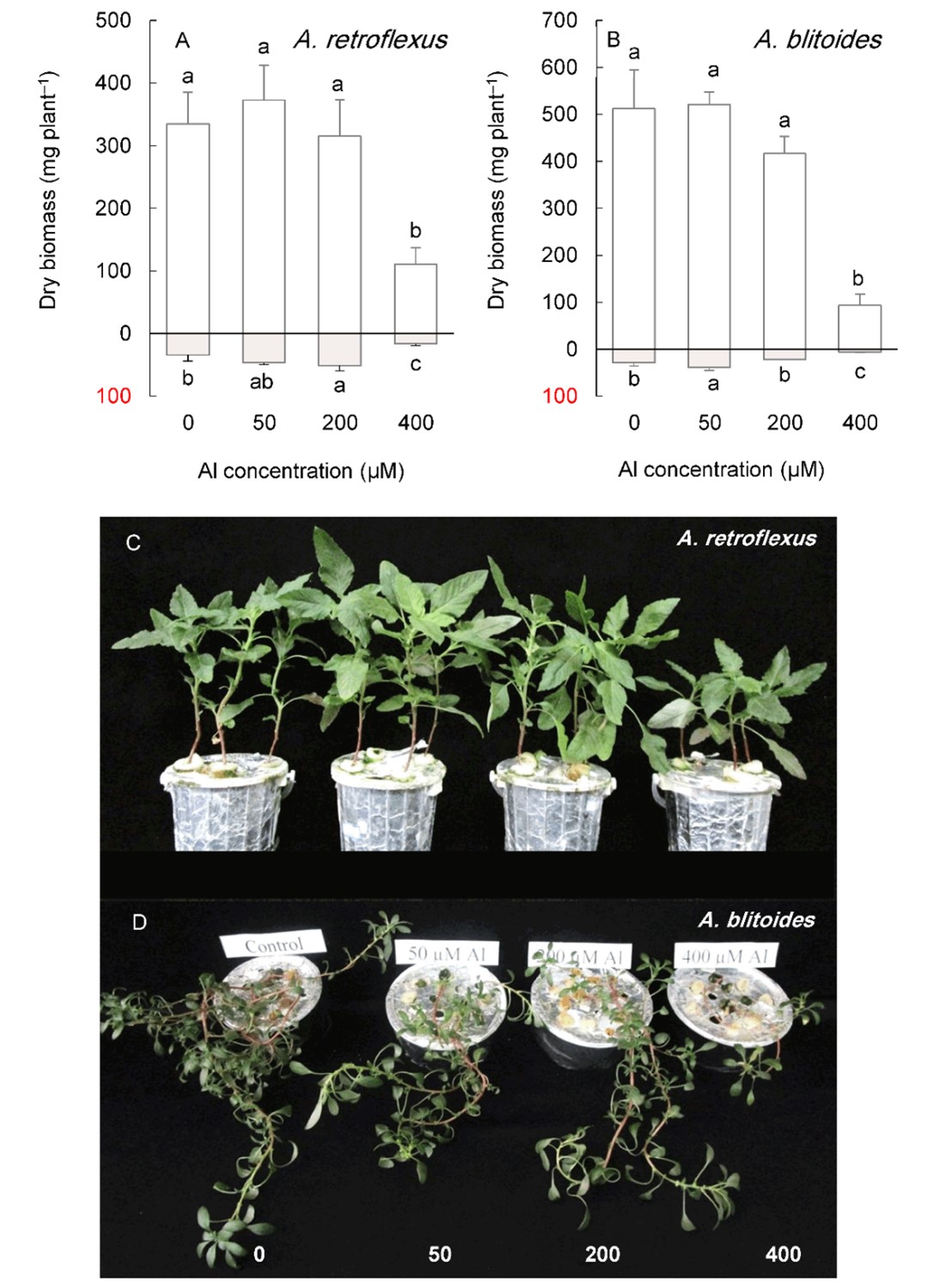
Fig. 5. Shoot (above the x-axis) and root (below the x-axis) biomass in Amaranthus retroflexus and A. blitoides grown for six weeks under different Al concentrations in the hydroponic medium. Data are mean values of 4 replicates ± standard deviation. Bars indicated by the same letters are not significantly different (P < 0.05). Pictures (C, D) show the plants at harvest.
In contrast, the root biomass increased under 50 or 200 µM Al, depending on the species, except for A. tricolor, whose root biomass did not change in this Al concentration range. The highest Al treatment level (400 µM) significantly reduced the shoot and root biomass of A. blitoides and A. retroflexus but did not affect the shoot biomass of A. cruentus and A. tricolor (Fig. 5 and Fig. 6).
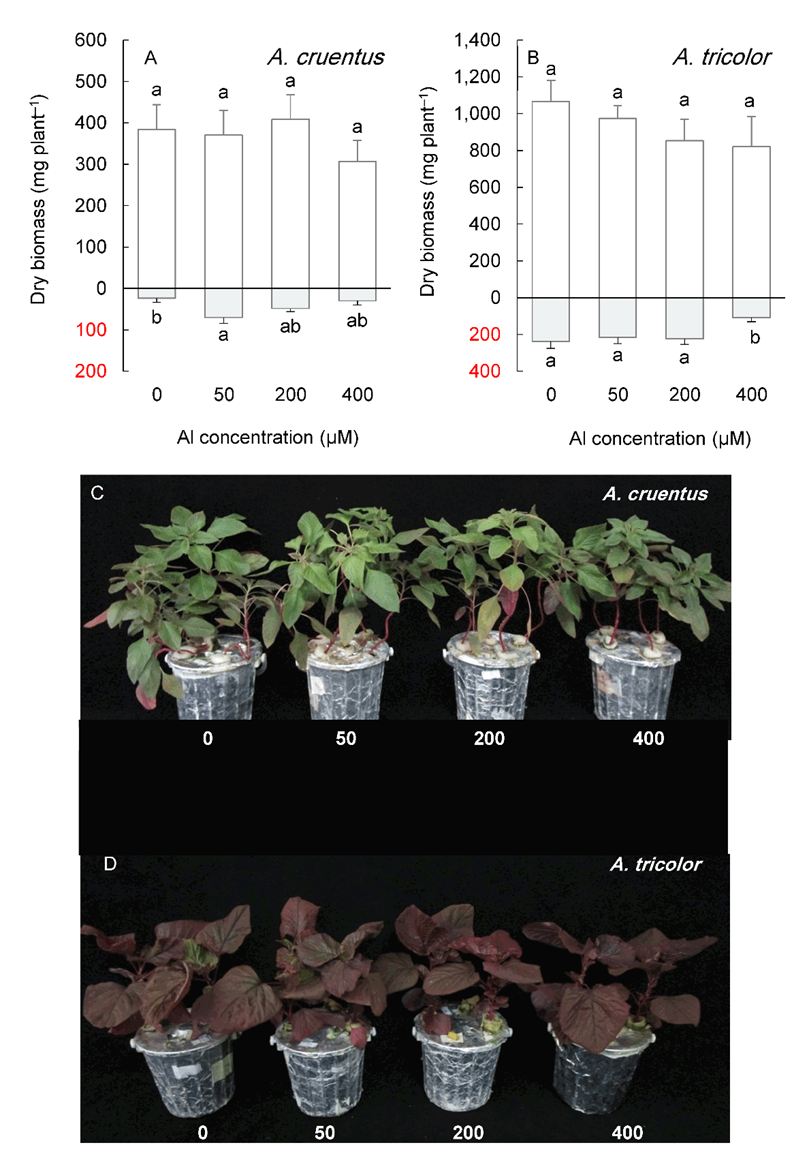
Fig. 6. Shoot (above the x-axis) and root (below the x-axis) biomass in Amaranthus cruentus (A) and A. tricolor (B) grown for six weeks under different Al concentrations in the hydroponic medium. Data are mean values of 4 replicates ± standard deviation. Bars indicated by the same letters are not significantly different (P < 0.05). Pictures (C, D) show the plants at harvest.
Al accumulation in the leaves increased as Al concentration in the medium increased (Fig. 7). The accumulation of Al was greater in older leaves than in younger leaves (P < 0.05). Al accumulation in the old leaves of plants treated with 400 µM Al exceeded the critical level established for Al accumulators in all studied species (Fig. 7). In the old leaves of A. blitoides and A. cruentus, Al accumulation of approximately 1 mg g –1 DW was also caused by 200 µM Al treatment (Fig. 7B, C).
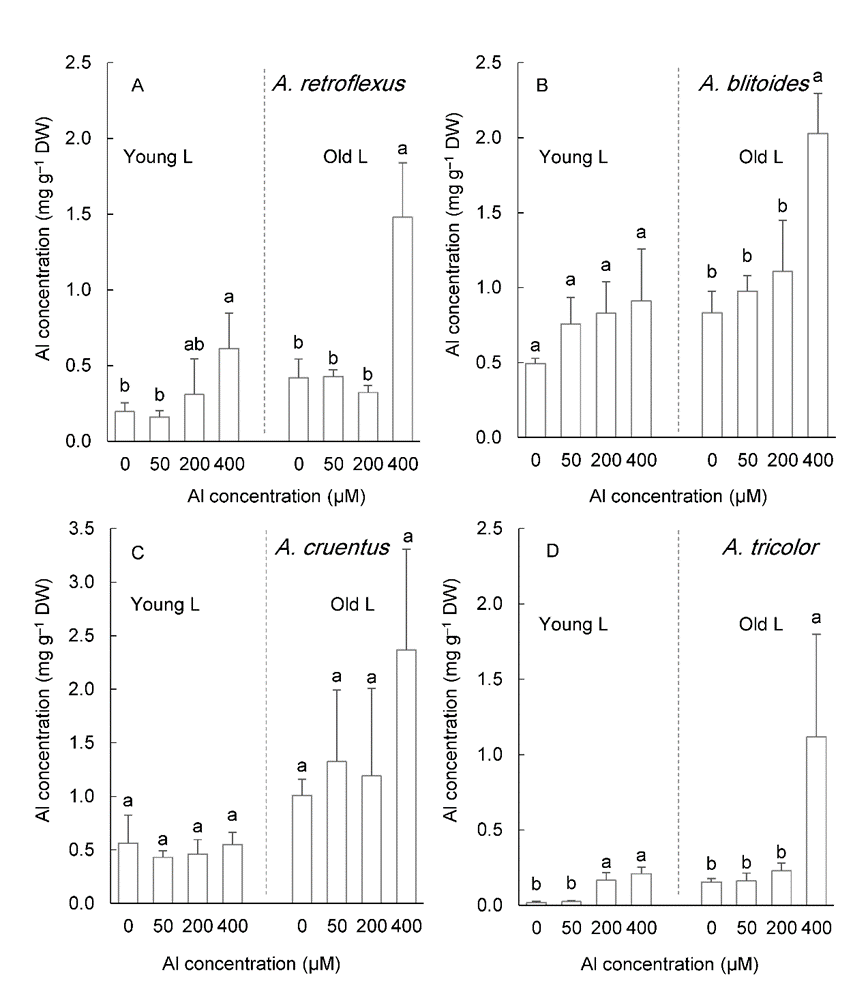
Fig. 7. Aluminum concentration in the young and old leaves in four Amaranthus species grown for six weeks under different Al concentrations in the hydroponic medium. Data are mean values of 4 replicates ± standard deviation. Bars indicated by the same letters are not significantly different ( P < 0.05).
Discussion
After three weeks of treatment with low Al concentrations (20-50 µM), the shoot biomass of all four Amaranthus species was significantly higher than that in control plants (Fig. 1 and Fig. 2). Even 200 µM Al increased the shoot biomass by 68% in A. tricolor. Growth stimulation by low concentrations of Al is relatively common in plant species adapted to acid soils and has been attributed to activation of photosynthesis and nitrogen assimilation, enhancement of nutrient uptake, alleviation of the toxic effects of H +, Fe 2+ and Mn 2+, activation of antioxidant defense and reduction of lignin (Bojórquez-Quintal et al. 2017, Hajiboland et al. 2022). In our work, only the activity of NR was improved among the physiological and biochemical parameters determined for the Amaranthus species. Nonetheless, higher nitrogen assimilation was not associated with a higher photosynthetic rate under these conditions, thus, its role in the growth promotion of these species remains unclear. Enhanced capacity for nutrient acquisition resulting from root growth stimulation observed in the experiment, could also be an additional mechanism of higher shoot biomass production in soil-grown plants.
In contrast to the root biomass, the improvement of shoot growth mediated by low Al concentrations disappeared under longer periods of time in all four studied species (Fig. 5 and Fig. 6). Such transient growth responses may be the consequence of the attenuation of the factors responsible for growth improvement under short-term Al exposure, but the underlying causes remain obscure. Regardless of the mechanisms, a significant growth improvement by Al in Amaranthus species during the early growth stage may be of great importance for the establishment of young plants in acidic soil conditions and is likely an advantage over species lacking such stimulating responses to Al.
The elongation rate of roots in response to Al exhibits three distinct patterns: growth stimulation by low Al level or short-term Al exposure due to alleviation of proton toxicity, consistent reduction of elongation due to Al 3+ toxicity, and induction of tolerance mechanisms and compensation of growth inhibition following a transient reduction (Barceló and Poschenrieder 2002). In this study, the taproot length, which may directly reflect the Al effect on the root elongation rate, was seen to be significantly stimulated in all Amaranthus species within a lower Al concentration range, the most prominent effect being observed at 20 µM Al, with a 39-95% increase depending on the species. However, a higher Al concentration (200 µM) only stimulated the taproot length in A. retroflexus.
At 400 µM Al concentration, a significant decrease in the tap-root length was observed in all studied species. Rhizotoxic Al 3+ inhibits root cell multiplication and expansion, thereby preventing its elongation (Doncheva et al. 2005). Recently, the involvement of the plant DNA damage pathway in the root response to Al has been demonstrated. Al-mediated root growth inhibition results from DNA damage and induction of repair mechanisms by the components of this pathway, which ultimately stop cell cycle progression (Pedroza‐Garcia et al. 2022).
The effect of Al on root growth was more pronounced when biomass data and total length were considered. Al concentrations (50-200 µM, depending on species) improved these parameters without causing an increase in tap root length (Fig. 3 and Fig. 4). Under these circumstances, more lateral root initials developed, resulting in an increase in total root length and root biomass. In susceptible species, this range of Al concentrations (12-60 µM free Al 3+ activity) is primarily toxic causing strong root growth inhibition (Poschenrieder et al. 2008). The Al-induced increase in ethylene and auxin, both of which are closely related to root growth, elicits significant adaptive responses to Al. These phytohormones are responsible for initiating more lateral roots in Al-tolerant species (Kopittke 2016), modifying the cell wall to reduce Al binding, and regulating the release of organic acids (Gao et al. 2022). Al-mediated improvement of root growth may be a crucial adaptive strategy for species native to acidic soils. A greater spatial availability may be vital for nutrient-poor, acidic soils typical of some regions, such as the Cerrado biome (Haridasan 2008). In addition, higher lateral root initiation primarily located in the upper portions of the roots in Amaranthus species may be more advantageous for plants in capturing the available nutrients in the topsoil. This soil profile is highly susceptible to leaching and nutrient loss, particularly of phosphorus (Withers et al. 2003).
The most Al 3+-sensitive portion of the root apex is the transition zone, which is the zone between the active cell division zone and the fast cell elongation zone (Sivaguru and Horst 1998, Poschenrieder et al. 2008). In the present study, the absence of hematoxylin staining in the 1.0-5.0 mm tip region (coincident with the transition and elongation zones) under 20-200 µM Al concentrations in Amaranthus species (except A. cruentus) suggests that these species are able to inhibit the binding of Al 3+ to the cell wall fractions in this zone, thereby preventing Al-induced inhibition of root elongation. As in other Al-tolerant species (Barceló and Poschenrieder 2002), excluding Al 3+ from the vulnerable root zones, i.e., apoplastic detoxification, was most likely accomplished via the exudation of chelating molecules, such as phenolics and organic acids. Excretion of oxalate and citrate in A. hypochondriacus (Fan et al. 2016) and phenolics in A. blitoides (Hajiboland, unpublished data) has been found in Al-exposed plants. Greater staining of the root axis under 50 µM Al in A. cruentus was associated with the least stimulation of tap root length of all the four species. Nevertheless, lower staining under 400 µM Al in A. retroflexus and A. tricolor was not correlated with less growth inhibition of total root length or taproot length in these species. Under these conditions, Al was likely entered into the root-tip symplast, resulting in less cell wall localization. The transporter mediating uptake of Al in a chelated form with organic acids (NIP1;2) has been identified in Arabidopsis, which is responsible for removing Al 3+ from the root cell wall (Wang et al. 2017).
In four Amaranthus species, higher growth stimulation at low Al concentrations (20-50 µM) was not necessarily associated with greater tolerance to the toxic Al concentration (400 µM). This is likely due to the involvement of two distinct mechanisms in the plant’s response to these varying Al concentrations. The growth improvement by low concentrations of metal ions (including Al 3+) is primarily attributable to enhanced tolerance to certain latent stress factors (the hormesis effect) (Poschenrieder et al. 2013). In contrast, tolerance to higher Al concentrations is due to specific mechanisms for sequestration and internal detoxification of Al 3+ (Bojórquez-Quintal et al. 2017, Hajiboland et al. 2022).
The root growth response has been considered a major criterion for the evaluation of the response of whole plants to Al (Barceló and Poschenrieder 2002). However, in our hydroponic study, root and shoot growth responded differently to Al (On-line Suppl. Fig. 2). In addition, although the beneficial effect of Al on root growth was observed in both short- and long-term treatments, no improvement in shoot biomass growth was detected in the long term Al treatments (Fig. 5 and Fig. 6). The absence of a correlation between shoot and root growth under both beneficial and toxic Al 3+ concentrations was likely the result of sufficient nutrient availability in the hydroponic medium, independent of the root surface area. However, root function may be crucial for the shoot response to Al for soil-grown plants due to its important role in nutrient acquisition from acid soil that tends to be deficient in essential macronutrients such as P, Ca, Mg, and K. The availability and concentration of these nutrients are major limiting factors for plant performance under acidic soil conditions (Kochian et al. 2004).
The biosynthesis of anthocyanins, a large group of water-soluble secondary plant metabolites, is stimulated by environmental stresses (Sakihama et al. 2002), indicating a functional relationship between their accumulation and stress adaptation. The formation of a complex between Al and anthocyanins has been shown under in vitro conditions (Moncada et al. 2003). The hydroxyl substituents in the B-ring of anthocyanins are bound to metal ions such as Al and form supramolecular complexes with colorless molecules (Quina et al. 2009), resulting in the flower’s color in Al-accumulator species such as hydrangea (Schreiber et al. 2011). However, the function of anthocyanins as chelating molecules for internal Al detoxification has not been addressed directly. Amaranthus species are abundant in anthocyanins (Paśko et al. 2009), however, their significance for Al chelation and detoxification has not yet been studied. In the present study, Al accumulation in the leaves of four Amaranthus species was not associated with correspondingly higher anthocyanin concentration. However, in the long-term experiment (Fig. 6), the red colored species ( A. cruentus and A. tricolor) exhibited much less inhibition of shoot growth under 400 µM Al compared to the green colored species ( A. retroflexus and A. blitoides) (Fig. 5). This may be indirect evidence for a role of anthocyanin in Al detoxification in the leaves.
After six weeks of growth under 400 µM Al, the concentration of Al in the old leaves of four Amaranthus species reached the critical Al accumulation level (1 mg g –1 DW). The Al concentrations in the old leaves of A. blitoides and A. cruentus were greater than this critical level even under 50 and 200 µM Al treatments, which suggests that these species have a higher accumulation capacity than others.
Conclusions
There is currently a lack of information regarding the tolerance of Amaranthus species to acid soils, particularly their Al accumulation capacity, despite their global distribution and adaptation to various soil and climate conditions. According to this study, all the four Amaranthus species grown in a hydroponic medium exhibited a high Al tolerance and Al accumulation capacity. Although the seeds of these species were collected from acid ( A. blitoides and A. retroflexus) or calcareous ( A. cruentus and A. tricolor) soils, there was no effect of habitat on their tolerance level to Al. This indicates that these species have the capacity to adapt to a broad range of soil conditions which is consistent with their cosmopolitan nature. However, for exploring an intraspecific variation driven by different soil pH, the comparison of plant populations from different habitats with contrasting soil pH is necessary.
According to our findings, these Amaranthus species could be used for the restoration and revegetation of acid-eroded soils, as model species for the physiological and molecular study of internal Al detoxification, and for phylogenetic studies by botanists interested in the origin of the Al accumulation trait in angiosperms. Considering the edible nature of some Amaranthus species, the Al concentration in the old leaves should be regarded as a potential human health risk.