Electrochemical sensor for determination of butylated hydroxyanisole in real samples using glassy carbon electrode modified by [Co(HL)2Cl2] nano-complex
Introduction
Butylated hydroxyanisole, a substituted phenolic compound, is one of the potent chemical antioxidants used as a preservative in food, food packaging, animal feed, cosmetics, pharmaceutical preparation, rubber and petroleum products. It is also used as a vitamin A stabilizer. BHA is added to food products to improve their stability, especially to prevent rancidity in products containing lipids or fats. BHA is exclusively used in food products because of its ability to remain active even at high temperatures while cooking or baking [1-3]. Although they are powerful in protecting product quality in food distribution, excess antioxidants added to food might produce toxicities or mutagenicities and, thus, endanger people's health [4,5]. Thus, it is necessary to reliably determine the amounts of BHA in food or other products.
Many methods, including spectrophotometry [6], gas chromatography [7], high-performance liquid chromatography [8], micellar electrokinetic capillary chromatography [9] and electrochemical methods [10,11] have been developed for the determination of BHA. Electrochemical sensors with excellent ability for the determination of electroactive substances, especially food samples, have been suggested as a powerful analytical tool in recent years [12-18]. Electrochemical sensors are fast, portable and affordable. However, in many cases, the detection limit was insufficient when traces of these compounds had to be determined. The results might also be irreproducible due to surface fouling, requiring high oxidation potentials and being time-consuming [19-26]. Chemically modified electrodes (CMEs) improve the sensitivity and selectivity of electrochemical analysis by improving the kinetics of the reaction via an electrocatalytic process at the CME surface [24-35].
Recently, more attention has been focused on the synthesis and application of nanoparticles (NPs), and on properties of NPs such as high electrical conductivity, high surface area, and chemical stability [36-40]. The NPs can be used to promote electron transfer reactions when it is used as electrode material in an electrochemical device. Therefore, the modification of the electrochemical interface by nanostructures is one of the recent approaches used extensively in the development of sensing platforms [41,43].
Schiff base ligands are among the most common coordinating ligands in coordination chemistry [44], through a direct forward condensation reaction between an amine and a carbonyl compound [45]. During the past few decades, considerable attention has been paid to the chemistry of metal complexes of Schiff bases containing nitrogen and other donor atoms [46]. This may be attributed to their stability, biological activity and potential applications in many fields, such as oxidation catalysis, electrochemistry [47], and biological properties, such as antibacterial, antifungal and antioxidant activities [48,49]. In general, the antibacterial and antifungal activities of Schiff bases are attributed to the presence of lone pair of electrons on the nitrogen atom and the electron-donating character of the double bond of the azomethine group [50]. Facile and inexpensive synthesis, allied with the wide range of structural and electronic features of Schiff bases and their coordination complexes, has increased interest in chemically modifying electrodes with these compounds. Therefore, the resulting electrodes have been used as sensors and/or probes in a variety of fields. All this interest has culminated in the development of low-cost sensors. In particular, the many structural and electronic properties of Schiff bases and their transition metal nano-complexes allow them and the analyte to establish different interactions, which improves sensor selectivity and sensitivity [51-56].
In this work, Schiff base ligand [HL] and its cobalt(II) complex (1) with (N-(2-hydoxynaphtalen)2-methyl aniline) Schiff base ligand were synthesized. The complex was characterized using spectroscopic techniques such as FT-IR, UV-Vis and elemental analysis. The crystal structure of title complex was determined by X-ray crystallography. The Schiff base cobalt nano-complex (2) was prepared by the sonochemical method. This synthesis was performed without the use of any surfactants. The nano-sized complex (2) was identified using XRD and SEM techniques. Also, we developed a voltammetric sensor based on [Co(HL)2Cl2] nano-complex modified glassy carbon electrode for the detection of BHA. Under optimal conditions, the sensor displayed excellent sensitivity, low detection limit and rapid response toward the analyte. Moreover, this developed sensor was applied in the determination of BHA in real samples successfully
Experimental
Materials and instrumentation
1H-NMR spectrum was accomplished with Bruker AVANCE3 3-300MHz spectrometer using DMSO-d6 as solvent and at room temperature (298 k). Fourier-transform infrared spectra were performed on a PerkinElmer FT-IR spectrophotometer model spectrum two with KBr discs in the range of 4000-400 cm-1. For elemental analyses of new compounds, a Costech ECS 4010 CHNS Elemental Analyzer was utilized. UV-Vis spectra were recorded in the range of 200–800 nm on Optizen view 2120UVplus Spectrophotometer ver1-2. Single crystal measurements were done with Rigaku OD Supernova, equipped with Atlas S2 CCD detector and Mo-Kα radiation from a micro-focused sealed X-ray tube. The data reduction, scaling and absorption correction were made in CrysAlis PRO. MCE was used for the visualization of electron density maps.
All the electrochemical measurements were carried out on a PGSTAT302N potentiostat/galvanostat Autolab consisting of a traditional three-electrode system: a bare or modified glassy carbon electrode (GCE) as the working electrode, an Ag/AgCl as the auxiliary electrode and a Pt wire as the counter electrode. Solution pH values were determined using a 713 pH meter combined with a glass electrode (Metrohm, Switzerland). BHA and other chemicals used were analytical grade and were purchased from Sigma Aldrich and Merck. Orthophosphoric acid was used to prepare the phosphate buffer solutions (PBSs), and sodium hydroxide was used to adjust the desired pH values (pH range between 2.0 and 9.0).
Synthesis of Schiff base ligand [HL]
The ligand (HL: N-(2-hydroxy-1-naphthylidene)-2-methyl aniline) was synthesized by adding a solution of 2-hydroxy-1-naphthaldehyde (0.17 g, 1 mmol) in 10 mL of ethanol to a solution of 2-mehyl aniline (0.11 ml, 1 mmol). Then, the mixture was refluxed for 6 h. The resulting yellow solution was placed at room temperature. After 24 h, yellow crystals of [HL] were formed. The Schiff base ligand was characterized by 1H-NMR, FT-IR, elemental analysis and single-crystal X-ray diffraction: mp:(154 °C);(yield: 88 %);(MW: 261.31); Anal. Calc. for [C18H15NO]: C82.73;H6.12; N 5.79; Found: C82.70; H6.09; N5.76. FTIR (KBr, cm-1), 3421ν (N-H), 1620 ν(C=N), 1161 ν(C-O), 1479 ν(C=C); 1H-NMR (300 MHz, DMSO d6, ppm) 9.61 (1H, s, CH=N), 16.05 (1H, OH), 2.40 (3H,CH3) [37].
Synthesis of complex (1)
The Schiff base ligand (HL: N-(2-hydroxy-1-naphthylidene)-2-methyl aniline) (0.26 g,1 mmol) was dissolved in 10 ml of methanol and to this yellow solution, a solution of [CoCl2.6H2O] (0.12 g, 0.5mmol) in 10 ml of methanol was added dropwise. The reaction mixture was refluxed for 6 h. The complex was precipitated, and the precipitate was filtered and dried at room temperature. Green crystals of [Co(HL)2Cl2] complex suitable for single crystal X-ray diffraction were obtained by the crystallization from the acetonitrile solution of the precipitate after two weeks. The complex was characterized by UV-Vis, FT- IR, elemental analysis and single-crystal X-ray diffraction (Scheme 1). mp:250 °C; (yield: 85 %); (MW: 652.5); Anal. Calc. for [C36H30Cl2CoN2O2]: C66.27; H4.63; N4.29; found: C66.32; H4.69; N4.27. FT-IR (KBr, cm-1), 1619 ν(C=N); 1146 ν(C-O), 535 ν(Co-O).
Synthesis of nanocomplex (2)
A solution of CoCl2.6H2O (0.12 g, 0.5 mmol) in methanol (10 mL) was positioned in a high-density ultrasonic probe for 10 min. Then to this solution, 10 ml of methanolic solution of Schiff base ligand (0.26 g, 1 mmol) was added dropwise. The resultant solution was then irradiated for 60 min at 60 °C with a power of 100 W. The obtained brown precipitate was filtered and dried in air. mp: 245 °C; (yield: 87 %); (MW: 652.5); Anal. Calc. for [C36H30CoCl2N2O2]: C 66.27; H 4.63; N 4.29; found: C 66.34; H, 4.69; N,4.27. FT-IR (KBr, cm-1): 1621 ν(C=N); 1147 ν(C-O), 534ν (Co-O).
Preparation of [Co(HL)2Cl2] nano-complex/GCE
The bare glassy carbon electrode was coated with [Co(HL)2Cl2] nano-complex according to the following simple procedure. 1 mg [Co(HL)2Cl2] nano-complex was dispersed in 1 mL aqueous solution within 50 min ultrasonication. Then, 4 μl of the prepared suspension was dropped on the surface of carbon working electrodes. It remains at room temperature until it becomes dry. The surface areas of the [Co(HL)2Cl2] nano-complex/GCE and the un-modified GCE were obtained by CV using 1 mM K3Fe(CN)6 at various scan rates. Using the Randles–Ševčik equation for [Co(HL)2Cl2] nano-complex/GCE, the electrode surface was found to be 0.109 cm2 which was about 3.5 times greater than un-modified GCE.
Results and discussion
Single crystal X-ray diffraction
Green crystals of [Co(HL)2Cl2] (1) were obtained by slow evaporation of an acetonitrile solution. Its structure was determined by single-crystal X-ray diffraction. The complex crystallized in the monoclinic system in space group C2/c with an asymmetric unit consisting of a metal cation in a special position, one chloride anion and one disordered HL ligand.Table 1 shows the crystallographic data for complex (1).
All hydrogen atoms of the strongly occupied part of ligands were discernible in difference Fourier maps and could be refined to reasonable geometry. According to common practice, H atoms bonded to C were kept in ideal positions with C–H = 0.96 Å, while positions bonded to N were refined with restrained bond length. In both cases, Uiso(H) was set to 1.2Ueq(C, N). All non-hydrogen atoms were refined using harmonic refinement. A disordered ligand was observed in both structures. Given the scale of disorder and numerous overlaps, we decided to use molecular refinement to model the disorders. Two models were used to allow for free rotation of the tolyl group. The occupancies of two positions were refined with the sum constrained to 1. Resulting occupancy ratios were 832(2):168(2). For further information on data collection and refinement, seeTable 1.
A view of compound (1) is shown inFigure 1. The occupied position is shown inFigure 2.
The cation in complex (1) is four-coordinate with two chloride anions and two phenolic oxygen atoms forming a slightly distorted tetrahedron. Only the oxygen atom of the neutral zwitterionic HL ligand is coordinated with the metal cation, while the presence of an azomethine proton denies the possibility of coordination via the nitrogen atom. A list of the most important bond distances and angles is reported inTable 2.
The coordination tetrahedron formed by chloride anions and the ligand with higher occupancy has only minor distortions from the ideal values with Co–Cl1 bond lengths at 2.2505(5) Å and Co–O1a bond lengths at 1.9618(16) Å. The bond angles are in the range from 104.82(6)° to 115.11(2)°. The coordination of weakly occupied oxygen atoms appears weaker compared to the oxygen atoms with higher occupancy, with Co–O1b bonding longer by 0.1602 Å compared to Co–O1a. This elongation brings the bond length closer to the length of Co–Cl1 bond, which should result in lower distortions from the ideal tetrahedron. Regardless of the similarities in the bond lengths, the distortions in the coordination tetrahedron formed with the weakly occupied ligand were even more pronounced, with the angles ranging from 99.06(19)° to 115.4(3)°.
FT-IR spectra
The FT-IR analysis data is listed inTable 3. The FT-IR spectrum of the free Schiff base ligand [HL] exhibits a band in 3421 due to ν(NH+) and a band in 1620 cm-1 due to ν(C=N) azomethine. A band at 1161 cm-1 is assigned to the ν(C-O) phenolic group. This band has been shifted to lower frequencies for both complex (1) and nano-complex (2), in the region of 1146-1147 cm-1, which indicates that both compounds are formed by the coordination of the oxygen atom of [HL] to the metal ion (Figure 3)s. FT-IR spectra of both complex (1) and nanocomplex (2) show weak bands at 535 cm-1 and 534 cm-1 that assign to (Co-O), and don't show any shift due to ν(C=N) and ν(NH+) that this display the azomethine group of [HL] doesn't participate in complex formation [57].
UV-Vis spectra
UV-Vis spectra of [HL], (1) and (2) in methanol solution contain different peaks related to the transition bands data. These absorption bands (nm) are listed inTable 2. The Schiff base ligand display two bands at 230 and 250 nm attributed to π →π* and a band at 330 nm that is assigned to n→π* transitions. In the electronic spectra of both (1) and (2), intra-ligand transitions (n→π* and π →π*) were shifted to another wavelength that this is due to the coordination of the metal to the ligands. Both of electronic spectra of complex (1) and nano-complex (2) showed three d-d absorption bands at 520-635 nm are assigned to the transition 4A2→4T2(F),4A2→4T1(F) and 4A2→4T1(P), respectively [58] (Figures 4 and5).
XRD and SEM
The XRD patterns of nano-sized Co(II) complex (2) and standard powder Co(II) complex (1) were obtained from single-crystal X-ray diffraction and are exhibited inFigure 6. The XRD pattern shows the crystalline phase and the nature of the complex. By investigating the location and intensity of the peaks of both patterns, it can be deduced that the diffraction angle in both complexes obtained by different methods is the same [59]. This indicates that the nano-sized complex (2) has a single crystalline phase that this phase is similar to that obtained by single-crystal X-ray diffraction [60]. The width of the diffraction peaks shows the nanocrystal complex (2) particles are of nanometer scales [61]. The particle means the size of the nanocrystal complex (2) was calculated using the Debye Scherrer equation. SEM images of the nanocrystal complex (2) are shown inFigure 7. The SEM photos also show the shape of the nanoparticles and the surface morphology of the nanocrystal complex. The average size diameter obtained from the Debye-Scherrer equation of nanocrystal complex (2) was approximately 56 nm.
Electrochemical behavior of BHA at the surface of various electrodes
According to our knowledge, the electrooxidation of BHA is closely related to the pH value of the solution (Scheme 2). So, the effect of pH was investigated using the differential pulse voltammetry (DPV) method. The results show that the oxidation peak current increased from pH 2.0 to 7.0, and then the current conversely decreased when the pH value increased from 7.0 to 9.0. According to obtained results, pH 7.0 was chosen as the best optimal experimental condition for other experiments.
The electrochemical behavior of BHA was investigated by linear sweep voltammetry (LSV). The linear sweep voltammograms obtained using the bare GCE and [Co(HL)2Cl2] nano-complex/GCE in 0.1 M PBS (pH 7.0) in the presence of 50.0 μM BHA are shown inFigure 8. At the bare CPE, a weak oxidation peak current (Ipa = 3.6 μA) could be seen at 0.57 V. In contrast, [Co(HL)2Cl2] nano-complex/GCE exhibited an enhanced sharp anodic peak current (Ipa =9.4 μA) at much lower overpotential Ep = 0.43 V. These results confirmed that the [Co(HL)2Cl2] nano-complex improved the sensitivity of the modified electrode by enhancing peak current and decreasing the overpotential of the oxidation of BHA.
Effect of scan rate on the determination of BHA at [Co(HL)2Cl2] nano-complex/GCE
The influence of the scan rate (ʋ) on the peak currents (Ipa) of BHA at [Co(HL)2Cl2] nano-complex/GCE was investigated by LSV.Figure 9 shows the voltammetric response of 50.0 μM BHA at [Co(HL)2Cl2] nano-complex/GCE at different scan rates in the range of 10 to 400 mV/s. The oxidation peak current of BHA increases linearly with increasing scan rate. A linear regression equation was obtained from the plot Ipa and vs. ʋ1/2 (square root of scan rate) as follows; Ipa (μA) = 1.3684 ʋ1/2 (mV/s)2 – 0.5184 (R2 = 0.9995) for the oxidation process, which indicates that the reaction of BHA at [Co(HL)2Cl2] nano-complex/GCE is diffusion controlled.
In order to obtain some information on the rate-determining step, we drew a Tafel plot (Figure 10) using the data from the rising part of the current-voltage curve recorded at a low scan rate of 10 mV s-1 for 50.0 μM BHA. The linearity of the E versus log I plot implies the intervention of the kinetics of the electrode process. The slope of this plot can be used to estimate the number of electrons transferred in the rate-determining step. According toFigure 10 inset, the Tafel slope for the linear part of the plot was estimated to be equal to 0.1316 V. The value of the Tafel slope indicates that the one-electron transfer process is the rate-limiting step, assuming a transfer coefficient (α) of about 0.55.
Chronoamperometric analysis
The analysis of chronoamperometry for BHA samples was performed by use of [Co(HL)2Cl2] nano-complex/GCE vs. Ag/AgCl/KCl (3.0 M) at 0.48 V. The chronoamperometric results of different concentrations of BHA in PBS (pH 7.0) are demonstrated inFigure 11. The Cottrell equation for the chronoamperometric analysis of electroactive moieties under mass transfer limited conditions is as follow:
where D represents the diffusion coefficient (cm2 s-1), and Cb is the applied bulk concentration (mol cm-3). Experimental results of I vs. t-1/2 were plotted inFigure 11A, with the best fits for different concentrations of BHA. The resulting slopes, corresponding to straight lines inFigure 11A, were then plotted against the concentration of BHA (Figure 11B). The mean value of D was determined to be 8.2 × 10-5 cm2/s according to the resulting slope and Cottrell equation.
Calibration curve
Because DPV commonly has a higher sensitivity than CV, the DPV technique was applied for the quantitative detection of BHA.Figure 12 shows the differential pulse voltammograms of BHA at various concentrations using [Co(HL)2Cl2] nano-complex/GCE. As seen, the oxidation peak currents of BHA enhance gradually by increasing its concentration. The oxidation peak currents (Ipa) show a good linear relationship with the concentrations of BHA ranging from 0.5 M to 150.0 μM. The linear equation is Ipa/μA = 0.171CBHA/μM + 0.4624 (R2 = 0.9994) (Figure 12 (inset)). Also, the limit of detection, Cm, of BHA was calculated using the following equation:
where, m is the slope of the calibration plot (0.171 μA/ μM) and Sb is the standard deviation of the blank response obtained from 15 replicate measurements of the blank solution. The detection limit for determination of BHA using this method of 0.12 μM was obtained.
Conclusions
We have reported the synthesis of a Schiff base ligand and a new Co(II) complex (1) . The [HL]and its cobalt(II) complex (1) were characterized by spectroscopic techniques and elemental analysis. The single crystal X-ray diffraction analysis of the complex showed that metal ions reacted with the ligand in a 1:2 molar ratio. In the formation of the complex, oxygen atoms of two ligands are coordinated to the metal ion, and tetrahedral geometry is formed around the metal ion with two chloride ions attached to it. Also, the nanocomplex (2) have been synthesized by the sonochemical process. The nanocomplex (2) was characterized via X-ray powder diffraction (XRD) and SEM. The XRD patterns indicated both (1) and (2) compounds prepared by different synthesis methods have the same crystal structure. A sensitive and fast voltammetric method to detect the BHA based on Co(HL)2Cl2] nano-complex (2) modified glassy carbon electrode was established. The voltammetric investigation demonstrates that electrooxidation of BHA at the surface of [Co(HL)2Cl2] nano-complex/GCE showed very distinct characteristics due to the presence of nanoparticles of [Co(HL)2Cl2] complex on the surface of electrode. [Co(HL)2Cl2] nano-complex/GCE exhibited good catalytic activity towards the oxidation of BHA over a linear range from 0.5 to 150.0 μM with an enhanced sensitivity of 0.171 μA/μM of BHA and favourable characteristics for the determination of BHA with a detection limit of 0.12 μM. Finally the proposed method was successfully applied in the determination of BHA in real samples with satisfactory results.
Acknowledgment
The authors sincerely thank the University of Sistan and Baluchestan for providing financial support for this work.
References
Freitas K.H.G.; Fatibello-Filho O. Simultaneous determination of butylated hydroxyanisole (BHA) and butylated hydroxytoluene (BHT) in food samples using a carbon composite electrode modified with Cu3(PO4)2 immobilized in polyester resin. Talanta 81 (2010) 1102-1108. https://doi.org/10.1016/j.talanta.2010.02.004 https://doi.org/10.1016/j.talanta.2010.02.004
Thomas D.; Rasheed Z.; Jagan J.S.; Kumar K.G. Study of kinetic parameters and development of a voltammetric sensor for the determination of butylated hydroxyanisole (BHA) in oil samples. Journal of Food Science and Technology 52 (2015) 6719-6726. https://doi.org/10.1007/s13197-015-1796-1 https://doi.org/10.1007/s13197-015-1796-1
Prabakar S.R.; Narayanan S.S. Surface modification of amine-functionalised graphite for preparation of cobalt hexacyanoferrate (CoHCF)-modified electrode: an amperometric sensor for determination of butylated hydroxyanisole (BHA). Analytical and Bioanalytical Chemistry 386 (2006) 2107-2115. https://doi.org/10.1007/s00216-006-0854-y https://doi.org/10.1007/s00216-006-0854-y
Cui M.; Liu S.; Lian W.; Li J.; Xu W.; Huang J. A molecularly-imprinted electrochemical sensor based on a graphene–Prussian blue composite-modified glassy carbon electrode for the detection of butylated hydroxyanisole in foodstuffs. Analyst 138 (2013) 5949-5955. https://doi.org/10.1039/C3AN01190A https://doi.org/10.1039/C3AN01190A
Rasheed Z.; Vikraman A.E.; Thomas D.; Jagan J.S.; Kumar K.G. Carbon-nanotube-based sensor for the determination of butylated hydroxyanisole in food samples. Food Analytical Methods 8 (2015) 213-221. https://doi.org/10.1007/s12161-014-9894-7 https://doi.org/10.1007/s12161-014-9894-7
Capitán-Vallvey L.F.; Valencia M.C.; Nicolás E.A. Simple resolution of butylated hydroxyanisole and n-propyl gallate in fatty foods and cosmetics samples by flow-injection solid-phase spectrophotometry. Journal of Food Science 68(5) (2003) 1595-1599. https://doi.org/10.1111/j.1365-2621.2003.tb12297.x https://doi.org/10.1111/j.1365-2621.2003.tb12297.x
Tombesi N.B.; Freije H. Application of solid-phase microextraction combined with gas chromatography–mass spectrometry to the determination of butylated hydroxytoluene in bottled drinking water. Journal of Chromatography A 963 (2002) 179-183. https://doi.org/10.1016/S0021-9673(02)00217-0 https://doi.org/10.1016/S0021-9673(02)00217-0
Sanches-Silva A.; Cruz J.M.; Sendón-Garcĺa R.; Paseiro-Losada P. Determination of butylated hydroxytoluene in food samples by high-performance liquid chromatography with ultraviolet detection and gas chromatography/mass spectrometry. Journal of AOAC International 90 (2007) 277-283. https://doi.org/10.1093/jaoac/90.1.277 https://doi.org/10.1093/jaoac/90.1.277
Guan Y.; Chu Q.; Fu L.; Wu T.; Ye J. Determination of phenolic antioxidants by micellar electrokinetic capillary chromatography with electrochemical detection. Food Chemistry 94 (2006) 157-162. https://doi.org/10.1016/j.foodchem.2005.01.015 https://doi.org/10.1016/j.foodchem.2005.01.015
Han S.; Ding Y.; Teng F.; Yao A.; Leng Q. Molecularly imprinted electrochemical sensor based on 3D-flower-like MoS2 decorated with silver nanoparticles for highly selective detection of butylated hydroxyanisole. Food Chemistry 387 (2022) 132899. https://doi.org/10.1016/j.foodchem.2022.132899 https://doi.org/10.1016/j.foodchem.2022.132899
Sangeetha N.S.; Narayanan S.S. Effective electrochemical detection of riboflavin and butylated hydroxy anisole based on azure A and nickel hexacyanoferrate framework on graphite electrode. Chemical Data Collections 30 (2020) 100544. https://doi.org/10.1016/j.cdc.2020.100544 https://doi.org/10.1016/j.cdc.2020.100544
Karimi-Maleh H.; Darabi R.; Shabani-Nooshabadi M.; Baghayeri M.; Karimi F.; Rouhi J.; Karaman C. Determination of D&C Red 33 and Patent Blue V Azo dyes using an impressive electrochemical sensor based on carbon paste electrode modified with ZIF-8/g-C3N4/Co and ionic liquid in mouthwash and toothpaste as real samples. Food and Chemical Toxicology (2022) 112907. https://doi.org/10.1016/j.fct.2022.112907 https://doi.org/10.1016/j.fct.2022.112907
Peyman H.; Roshanfekr H.; Babakhanian A.; Jafari H. PVC Membrane Electrode Modified by Lawson as Synthetic Derivative Ionophore for Determination of Cadmium in Alloy and Wastewater. Chemical Methodologie 5(5) (2020) 446-453. http://www.chemmethod.com/article_135266.html
Beitollahi H.; Mohammadi S.Z.; Safaei M.; Tajik S. Applications of electrochemical sensors and biosensors based on modified screen-printed electrodes: a review. Analytical Methods 12 (2020) 1547-1560. https://doi.org/10.1039/C9AY02598G https://doi.org/10.1039/C9AY02598G
Saghiri S.; Ebrahimi M.; Bozorgmehr M.R. NiO nanoparticle/1-hexyl-3-methylimidazolium hexafluorophosphate composite for amplification of epinephrine electrochemical sensor. Asian Journal of Nanosciences and Materials 4 (2021) 46-52. https://doi.org/10.26655/AJNANOMAT.2021.1.4 https://doi.org/10.26655/AJNANOMAT.2021.1.4
Mustafa Y.F.; Chehardoli G.; Habibzadeh S.; Arzehgar Z. Electrochemical detection of sulfite in food samples. Journal of Electrochemical Science and Engineering 12 (2022) 1061-1079. https://doi.org/10.5599/jese.1555 https://doi.org/10.5599/jese.1555
Eren T.; Atar N.; Yola M. L.; Karimi-Maleh H. A sensitive molecularly imprinted polymer based quartz crystal microbalance nanosensor for selective determination of lovastatin in red yeast rice. Food Chemistry 185 (2015) 430-436. https://doi.org/10.1016/j.foodchem.2015.03.153 https://doi.org/10.1016/j.foodchem.2015.03.153
Sengar M.S.; Saxena S.; Satsangee S.P.; Jain R. Silver Nanoparticles Decorated Functionalized Multiwalled Carbon Nanotubes Modified Screen Printed Sensor for Voltammetric Determination of Butorphanol. Journal of Applied Organometallic Chemistry 1 (2021) 95-108. https://doi.org/10.22034/jaoc.2021.289344.1023 https://doi.org/10.22034/jaoc.2021.289344.1023
Mazloum-Ardakani M.; Beitollahi H.; Ganjipour B.; Naeimi H. Novel carbon nanotube paste electrode for simultaneous determination of norepinephrine, uric acid and d-penicillamine. International Journal of Electrochemical Science 5 (2010) 531-546.
Lohrasbi-Nejad A. Electrochemical strategies for detection of diazinon. Journal of Electrochemical Science and Engineering 12 (2022) 1041-1059. https://doi.org/10.5599/jese.1379 https://doi.org/10.5599/jese.1379
Karimi-Maleh H.; Shojaei A.F.; Tabatabaeian K.; Karimi F.; Shakeri S.; Moradi R. Simultaneous determination of 6-mercaptopruine, 6-thioguanine and dasatinib as three important anticancer drugs using nanostructure voltammetric sensor employing Pt/MWCNTs and 1-butyl-3-methylimidazolium hexafluoro phosphate. Biosensors and Bioelectronics 86 (2016) 879-884. https://doi.org/10.1016/j.bios.2016.07.086 https://doi.org/10.1016/j.bios.2016.07.086
Mehdizadeh Z.; Shahidi S.; Ghorbani-HasanSaraei A.; Limooei M.; Bijad M. Monitoring of Amaranth in Drinking Samples using Voltammetric Amplified Electroanalytical Sensor. Chemical Methodologies 6 (2022) 246-252. https://doi.org/10.22034/chemm.2022.324073.1423 https://doi.org/10.22034/chemm.2022.324073.1423
Tajik S.; Beitollahi H.; Nejad F.G.; Shoaie I.S.; Khalilzadeh M.A.; Asl M.S.; Shokouhimehr M. Recent developments in conducting polymers: Applications for electrochemistry. RSC advances 10(62) (2020) 37834-37856. https://doi.org/10.1039/D0RA06160C https://doi.org/10.1039/D0RA06160C
Shamsi A.; Ahour F. Electrochemical Sensing of Thioridazine in Human Serum Samples Using Modified Glassy Carbon Electrode. Advanced Journal of Chemistry-Section A 4 (2021) 22-31. https://doi.org/10.22034/ajca.2020.252025.1215 https://doi.org/10.22034/ajca.2020.252025.1215
Jahani P.M. Flower-like MoS2 screen-printed electrode based sensor for the sensitive detection of sunset yellow FCF in food samples. Journal of Electrochemical Science and Engineering 12 (2022) 1099-1109. https://doi.org/10.5599/jese.1413 https://doi.org/10.5599/jese.1413
Alavi-Tabari S.A.; Khalilzadeh M. A.; Karimi-Maleh H. Simultaneous determination of doxorubicin and dasatinib as two breast anticancer drugs uses an amplified sensor with ionic liquid and ZnO nanoparticle. Journal of Electroanalytical Chemistry 811 (2018) 84-88. https://doi.org/10.1016/j.jelechem.2018.01.034 https://doi.org/10.1016/j.jelechem.2018.01.034
Azimi S.; Amiri M.; Imanzadeh H.; Bezaatpour A. Fe3O4@SiO2-NH2/CoSB Modified Carbon Paste Electrode for Simultaneous Detection of Acetaminophen and Chlorpheniramine. Advanced Journal of Chemistry-Section A 4 (2021) 152-164. https://doi.org/10.22034/ajca.2021.275901.1246 https://doi.org/10.22034/ajca.2021.275901.1246
Tajik S.; Taher M.A.; Beitollahi H. First report for simultaneous determination of methyldopa and hydrochlorothiazide using a nanostructured based electrochemical sensor. Journal of Electroanalytical Chemistry 704 (2013) 137-144. https://doi.org/10.1016/j.jelechem.2013.07.008 https://doi.org/10.1016/j.jelechem.2013.07.008
Vardini M.; Abbasi N.; Kaviani A.; Ahmadi M.; Karimi E. Graphite Electrode Potentiometric Sensor Modified by Surface Imprinted Silica Gel to Measure Valproic Acid. Chemical Methodologies 6 (2022) 398-408. http://www.chemmethod.com/article_147528.html
Ariavand S.; Ebrahimi M.; Foladi E. Design and Construction of a Novel and an Efficient Potentiometric Sensor for Determination of Sodium Ion in Urban Water Samples. Chemical Methodologies 6(11) (2022) 886-904. https://doi.org/10.22034/chemm.2022.348712.1567 https://doi.org/10.22034/chemm.2022.348712.1567
Karimi-Maleh H.; Sheikhshoaie M.; Sheikhshoaie I.; Ranjbar M.; Alizadeh J.; Maxakato N.W.; Abbaspourrad A. A novel electrochemical epinine sensor using amplified CuO nanoparticles and an-hexyl-3-methylimidazolium hexafluorophosphate electrode. New Journal of Chemistry 43 (2019), 2362-2367. https://doi.org/10.1039/C8NJ05581E https://doi.org/10.1039/C8NJ05581E
Mohabis R.M.; Fazeli F.; Amini I.; Azizkhani V. An overview of recent advances in the detection of ascorbic acid by electrochemical techniques. Journal of Electrochemical Science and Engineering 12 (2022) 1081-1098. https://doi.org/10.5599/jese.1561 https://doi.org/10.5599/jese.1561
Tajik S.; Taher M.A.; Beitollahi H. Simultaneous determination of droxidopa and carbidopa using a carbon nanotubes paste electrode. Sensors and Actuators B: Chemical 188 (2013) 923-930. https://doi.org/10.1016/j.snb.2013.07.085 https://doi.org/10.1016/j.snb.2013.07.085
Hosseini Fakhrabad A.; Sanavi Khoshnood R.; Abedi M.R.; Ebrahimi M. Fabrication a composite carbon paste electrodes (CPEs) modified with multi-wall carbon nano-tubes (MWCNTs/N, N-Bis (salicyliden)-1,3-propandiamine) for determination of lanthanum (III). Eurasian Chemical Communications 3 (2021) 627-634. https://doi.org/10.22034/ecc.2021.288271.1182 https://doi.org/10.22034/ecc.2021.288271.1182
Harismah K.; Mirzaei M.; Dai M.; Roshandel Z.; Salarrezaei E. In silico investigation of nanocarbon biosensors for diagnosis of COVID-19. Eurasian Chemical Communications 3(2) (2021) 95-102. https://doi.org/10.22034/ecc.2021.267226.1120 https://doi.org/10.22034/ecc.2021.267226.1120
Karimi-Maleh H.; Karaman C.; Karaman O.; Karimi F.; Vasseghian Y.; Fu L.; Mirabi A. Nanochemistry approach for the fabrication of Fe and N co-decorated biomass-derived activated carbon frameworks: a promising oxygen reduction reaction electrocatalyst in neutral media. Journal of Nanostructure in Chemistry (2022) 1-11. https://doi.org/10.1007/s40097-022-00492-3 https://doi.org/10.1007/s40097-022-00492-3
Dehno Khalaji A.; Machek P.; Jarosova M. α-Fe2O3 Nanoparticles: Synthesis, Characterization, Magnetic Properties and Photocatalytic Degradation of Methyl Orange. Advanced Journal of Chemistry-Section A 4(4) (2021) 317-326. https://doi.org/10.22034/ajca.2021.292396.1268 https://doi.org/10.22034/ajca.2021.292396.1268
Hajinasir R.; Esmaeili Jadidi M. Synthesis of ZnO nanoparticles via flaxseed aqueous extract. Journal of Applied Organometallic Chemistry 2(2) (2022) 101-108. https://doi.org/10.22034/jaoc.2022.154805 https://doi.org/10.22034/jaoc.2022.154805
Alidadykhoh M.; Pyman H.; Roshanfekr H. Application of a New Polymer Agcl Nanoparticles Coated Polyethylene Terephetalat [PET] as Adsorbent for Removal and Electrochemical Determination of Methylene Blue Dye. Chemical Methodologie 5(2) (2021) 96-106. https://doi.org/10.22034/chemm.2021.119677 https://doi.org/10.22034/chemm.2021.119677
Koohzadi N.; Rezayati Zad Z. A Highly Sensitive Colorimetric Determination of Paraquat by Silver Nanoparticles. Advanced Journal of Chemistry-Section B: Natural Products and Medical Chemistry 3(4) (2021) 311-322. https://doi.org/10.22034/ajcb.2021.302596.1092 https://doi.org/10.22034/ajcb.2021.302596.1092
Miraki M.; Karimi-Maleh H.; Taher M.A.; Cheraghi S.; Karimi F.; Agarwal S.; Gupta V.K. Voltammetric amplified platform based on ionic liquid/NiO nanocomposite for determination of benserazide and levodopa. Journal of Molecular Liquids 278 (2019) 672-676. https://doi.org/10.1016/j.molliq.2019.01.081 https://doi.org/10.1016/j.molliq.2019.01.081
Beitollahi H.; Sheikhshoaie I. Novel nanostructure-based electrochemical sensor for simultaneous determination of dopamine and acetaminophen. Materials Science and Engineering: C 32(2) (2012) 375-380. https://doi.org/10.1016/j.msec.2011.11.009 https://doi.org/10.1016/j.msec.2011.11.009
Bijad M.; Hojjati-Najafabadi A.; Asari-Bami H.; Habibzadeh S.; Amini I.; Fazeli F. An overview of modified sensors with focus on electrochemical sensing of sulfite in food samples. Eurasian Chemical Communications 3(2) (2021) 116-138. https://doi.org/10.22034/ecc.2021.268819.1122 https://doi.org/10.22034/ecc.2021.268819.1122
Sahraei A.; Kargar H.; Hakimi M.; Tahir M.N. Distorted square-antiprism geometry of new zirconium (IV) Schiff base complexes: Synthesis, spectral characterization, crystal structure and investigation of biological properties. Journal of Molecular Structure 1149 (2017) 576-584. https://doi.org/10.1016/j.molstruc.2017.08.022 https://doi.org/10.1016/j.molstruc.2017.08.022
Trzesowska-Kruszynska A. Copper complex of glycine Schiff base: In situ ligand synthesis, structure, spectral, and thermal properties. Journal of Molecular Structure 1017 (2012) 72-78. https://doi.org/10.1016/j.molstruc.2012.03.003 https://doi.org/10.1016/j.molstruc.2012.03.003
Joseph J.; Rani G.A.B. Metal based SOD mimetic therapeutic agents: Synthesis, characterization and biochemical studies of metal complexes. Arabian Journal of Chemistry 10 (2017) S1963-S1972. https://doi.org/10.1016/j.arabjc.2013.07.024 https://doi.org/10.1016/j.arabjc.2013.07.024
Liu X.; Hamon J.R. Recent developments in penta-, hexa-and heptadentate Schiff base ligands and their metal complexes. Coordination Chemistry Reviews 389 (2019) 94-118. https://doi.org/10.1016/j.ccr.2019.03.010 https://doi.org/10.1016/j.ccr.2019.03.010
Kaya S.; Erkan S.; Karakaş D. Computational investigation of molecular structures, spectroscopic properties and antitumor-antibacterial activities of some Schiff bases. Spectrochimica Acta Part A: Molecular and Biomolecular Spectroscopy 244 (2021) 118829. https://doi.org/10.1016/j.saa.2020.118829 https://doi.org/10.1016/j.saa.2020.118829
Abu-Dief A.M.; Mohamed I.M. A review on versatile applications of transition metal complexes incorporating Schiff bases. Beni-suef University Journal of Basic and Applied Sciences 4(2) (2015) 119-133. https://doi.org/10.1016/j.bjbas.2015.05.004 https://doi.org/10.1016/j.bjbas.2015.05.004
Dailami S.A.; Onakpa S.A.; Funtua M.A. Fe (III) and Cr (III) Complexes with Phenolic Schiff Base: Synthesis, Physico-Chemical Characterisation, Antimicrobial and Antioxidant Studies. IOSR Journal of Applied Chemistry 9(9) (2016) 18-23.
Tozzo E.; Romera S.; dos Santos M.P.; Muraro M.; Regina H.D.A.; Liao L.M.; Dockal E.R. Synthesis, spectral studies and X-ray crystal structure of N, N′-(±)-trans-1, 2-cyclohexylenebis (3-ethoxysalicylideneamine) H2 (t-3-EtOsalchxn). Journal of Molecular Structure 876 (2008) 110-120. https://doi.org/10.1016/j.molstruc.2007.05.043 https://doi.org/10.1016/j.molstruc.2007.05.043
Masoudi M.; Behzad M.; Arab A.; Tarahhomi A.; Rudbari H.A.; Bruno G. Crystal structures, DFT calculations and Hirshfeld surface analyses of three new cobalt (III) Schiff base complexes derived from meso-1, 2-diphenyl-1, 2-ethylenediamine. Journal of Molecular Structure 1122 (2016) 123-133. https://doi.org/10.1016/j.molstruc.2016.05.092 https://doi.org/10.1016/j.molstruc.2016.05.092
Cozzi P.G. Metal–Salen Schiff base complexes in catalysis: practical aspects. Chemical Society Reviews 33 (2004) 410-421. https://doi.org/10.1039/B307853C https://doi.org/10.1039/B307853C
Fatibello-Filho O.; Dockal E.R.; Marcolino-Junior L.H.; Teixeira M.F. Electrochemical modified electrodes based on metal-salen complexes. Analytical letters 40 (2007) 1825-1852. https://doi.org/10.1080/00032710701487122 https://doi.org/10.1080/00032710701487122
Al Zoubi W.; Al Mohanna N. Membrane sensors based on Schiff bases as chelating ionophores–A review. Spectrochimica Acta Part A: Molecular and Biomolecular Spectroscopy 132 (2014) 854-870. https://doi.org/10.1016/j.saa.2014.04.176 https://doi.org/10.1016/j.saa.2014.04.176
Oiye É.N.; Ribeiro M.F.M.; Katayama J.M.T.; Tadini M.C.; Balbino M.A.; Eleotério I.C.; de Oliveira M.F. Electrochemical sensors containing Schiff bases and their transition metal complexes to detect analytes of forensic, pharmaceutical and environmental interest. A review. Critical Reviews in Analytical Chemistry 49(6) (2019) 488-509. https://doi.org/10.1080/10408347.2018.1561242 https://doi.org/10.1080/10408347.2018.1561242
Taha R.H.; El-Shafiey Z.A.; Salman A.A.; El-Fakharany E.M.; Mansour M.M. Synthesis and characterrization of newly synthesized Schiff base ligand and its metal complexes as potent anticancer. Journal of Molecular Structure 1181 (2019) 536-545. https://doi.org/10.1016/j.molstruc.2018.12.055 https://doi.org/10.1016/j.molstruc.2018.12.055
Yousif E.; Majeed A.; Al-Sammarrae K.; Salih N.; Salimon J.; Abdullah B. Metal complexes of Schiff base: preparation, characterization and antibacterial activity. Arabian Journal of Chemistry 10 (2017) S1639-S1644. https://doi.org/10.1016/j.arabjc.2013.06.006 https://doi.org/10.1016/j.arabjc.2013.06.006
Biabani M.; Saravani H.; Eigner V.; Dusek M. A novel coordination polymer of Cd (II) based on 2-mercaptopyrimidine: sonochemical synthesis, characterization, and antibacterial properties. Journal of Molecular Structure 1166 (2018) 470-478. https://doi.org/10.1016/j.molstruc.2018.04.054 https://doi.org/10.1016/j.molstruc.2018.04.054
Sadeghzadeh H.; Morsali A. Sonochemical synthesis and characterization of nano-belt lead (II) coordination polymer: new precursor to produce pure phase nano-sized lead (II) oxide. Ultrasonics Sonochemistry 18(1) (2011) 80-84. https://doi.org/10.1016/j.ultsonch.2010.01.011 https://doi.org/10.1016/j.ultsonch.2010.01.011
Saif M.; El-Shafiy H.F.; Mashaly M.M.; Eid M.F.; Nabeel A.I.; Fouad R. Synthesis, characterization, and antioxidant/cytotoxic activity of new chromone Schiff base nano-complexes of Zn (II), Cu (II), Ni (II) and Co (II). Journal of Molecular Structure 1118 (2016) 75-82. https://doi.org/10.1016/j.molstruc.2016.03.060 https://doi.org/10.1016/j.molstruc.2016.03.060
Floating objects
Scheme1. Synthetic route for complex (1).
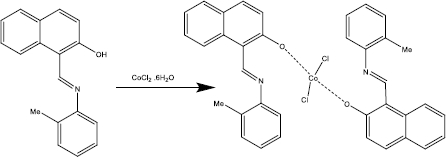
Scheme 2. The proposed mechanism for the oxidation of BHA at the [Co(HL)2Cl2] nano-complex/GCE.
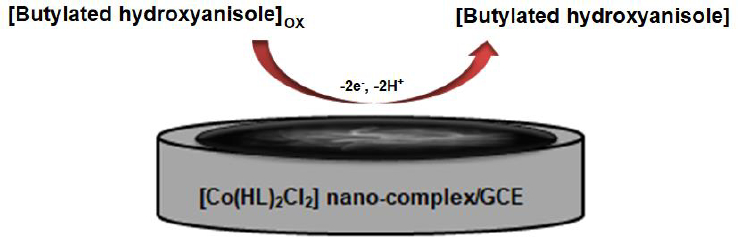
Figure 1. The labeled diagram of compound (1). Thermal ellipsoids are at 50% probability level symmetry code: (i) 1-x, y, 0.5-z.
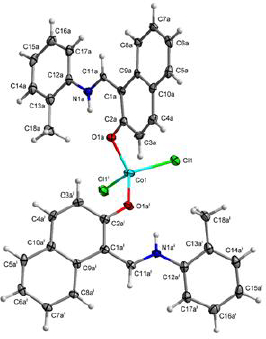
Figure 2. Disorder in complex (1), weakly occupied position depicted in blue with dashed bonds.
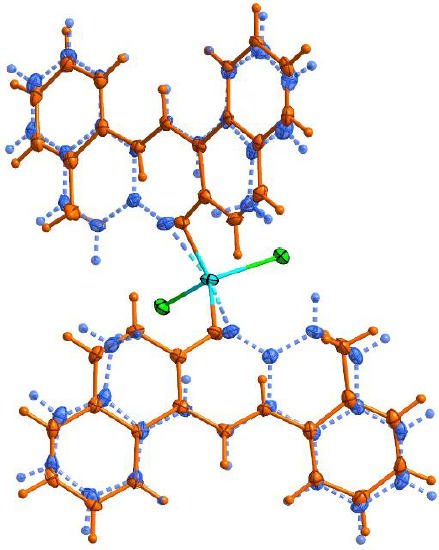
Figure 3. The FT-IR spectra of the complex (1) and its nanoparticles (a2)
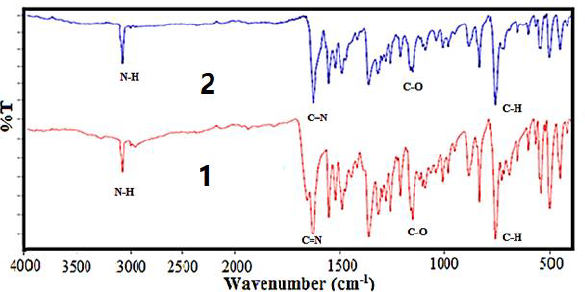
Figure 4. The spectrum of UV-Vis of (1) in methanol.
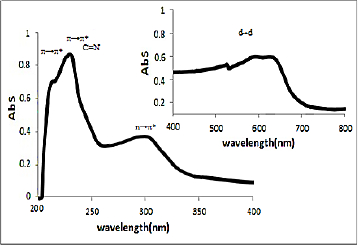
Figure 5. The spectrum of UV-Vis of (2) in methanol.
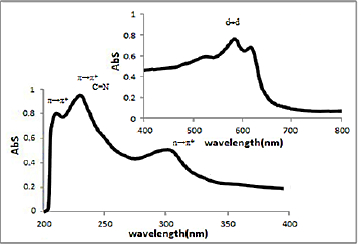
Figure 6. The XRD patterns of a: standard powder Co(II) complex (1) b: nano-sized Co(II) complex (2)
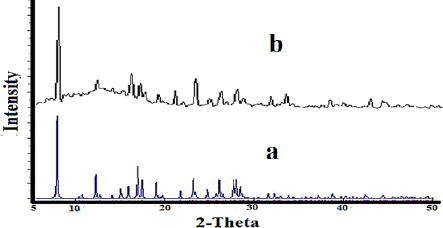
Figure 7. SEM images of the nanocrystal complex (2)
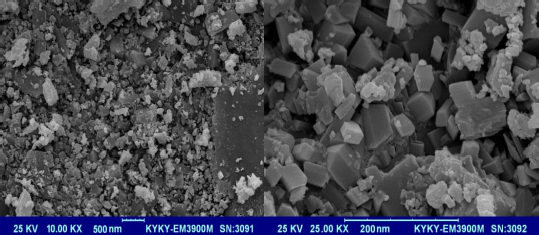
Figure 8. The linear sweep voltammograms of (a) bare GCE and (b) [Co(HL)2Cl2] nano-complex/GCE in 0.1 M PBS (pH 7.0) in the presence of 50.0 μM BHA at the scan rate 50 mVs-1.
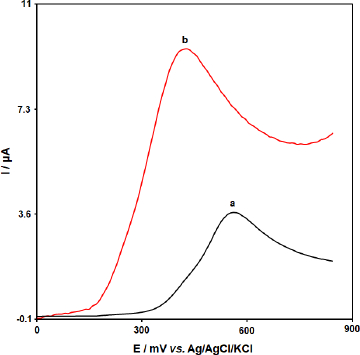
Figure 9. Linear sweep voltammograms of [Co(HL)2Cl2] nano-complex/GCE in 0.1 M PBS (pH 7.0) containing 50.0 μM BHA at various scan rates; 1-6 correspond to 10, 50, 100, 200, 300, and 400 mV s-1, respectively. Inset: variation of anodic peak current vs. ν1/2.
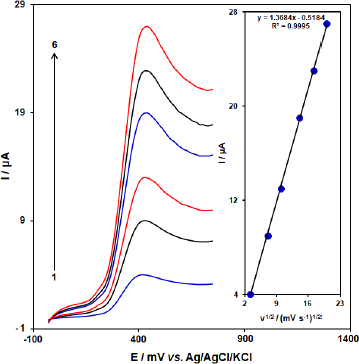
Figure 10. Linear sweep voltammograms response for 50.0 μM BHA with 10 mVs-1 scan rate. Inset: The Tafel plot derived from the rising part or the corresponding voltammogram.
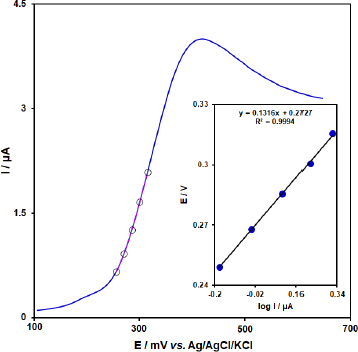
Figure 11. Chronoamperograms obtained at [Co(HL)2Cl2] nano-complex/GCE in 0.1 M PBS (pH 7.0) for different concentration of BHA. The 1-4 correspond to 0.1, 0.5, 0.9, and 2.0 mM of BHA. Insets: (A) Plots of I vs. t-1/2 obtained from chronoamperograms 1-4. (B) Plot of the slope of the straight lines against BHA concentration.
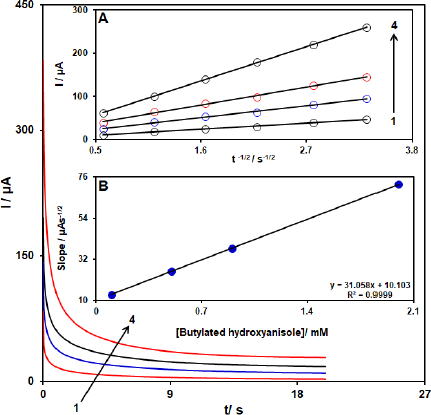
Figure 12. DPVs of [Co(HL)2Cl2] nano-complex/GCE in 0.1 M (pH 7.0) containing different concentrations of BHA. Numbers 1–7 correspond to 0.5, 5.0, 15.0, 45.0, 75.0, 100.0, and 150.0 μM of BHA. Inset: plot of the electrocatalytic peak current as a function of BHA concentration in the range of 0.5-150.0 μM.
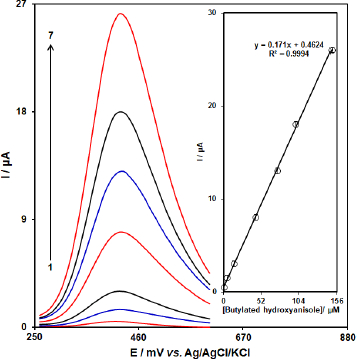
Table 1. Crystallographic data and refinement parameters for complex (1)
Compound | (1) |
---|
Formula | C36H30Cl2CoN2O2 |
Formula weight (g mol-1) | 652.5 |
λ (Å) | 0.71073 |
crystal system | monoclinic |
space group | C 2/c |
Hall group | -C 2yc |
a (Å) | 24.3845(7) |
b (Å) | 7.5848(3) |
c (Å) | 17.9596(9) |
β (°) | 114.763(3) |
T (K) | 95 |
V (Å3) | 3016.2(2) |
Z | 4 |
Dcalc (g cm-3) | 1.437 |
μ (mm-1) | 0.784 |
F(000) | 1348 |
h,k,lmax | 33,10,15 |
θmax (°) | 29.34 |
Measured refl. | 7862 |
Indep. refl. (Rint) | 3594 (0.018) |
Obs. refl. (I>3σ(I)) | 3110 |
R1(obs) | 0.0343 |
wR2(all) | 0.0998 |
S | 1.510 |
Parameters | 211 |
Δρmax, Δρmin (e Å-3) | 0.35, -0.36 |
CCDC | 1995453 |
Table 2. Selected bond lengths and angles in structures (1) and (2)
X–Y | (1) X–Y (Å) | X–Y–Z | (1) X–Y–Z (°) |
---|
Co–Cl1 | 2.2505(5) | Cl1–Co–Cl1i | 115.11(2) |
Co–Cl1i | 2.2505(5) | Cl1–Co–O1a | 104.82(6) |
Co–O1a | 1.9618(16) | Cl1–Co–O1ai | 110.14(5) |
Co–O1ai | 1.9618(16) | Cl1i–Co–O1a | 110.14(5) |
Co–O1b | 2.122(16) | Cl1i–Co–O1ai | 104.82(6) |
Co–O1bi | 2.122(16) | O1a–Co–O1ai | 111.97(7) |
| | Cl1–Co–O1b | 114.6(2) |
| | Cl1–Co–O1bi | 99.06(19) |
| | Cl1i–Co–O1b | 99.06(19) |
| | Cl1i–Co–O1bi | 114.6(2) |
| | O1b–Co–O1bi | 115.4(3) |
Table 3. Selected IR frequencies (cm-1) of [HL], (1) and (2).
compound | ν(N-H) | ν(C=N) | ν(C-O) | ν(Co-O) |
HL | 3421 | 1620 | 1161 | - |
Co(HL)2Cl2 (1) | 3425 | 1619 | 1146 | 535 |
Co(HL)2Cl2 (2) | 3425 | 1621 | 1147 | 534 |