Introduction
Infections caused by bacteria remain one of the major global health problems. Antibiotics have been used for bacterial infection treatments. However, selective pressure, mutation, inappropriate use of antibiotics, and inadequate diagnosis have resulted in the rapid emergence and proliferation of multi-drug-resistant bacterial strains [1]. Multidrug-resistant (MDR) bacteria are a worldwide public health problem that increases morbidity and mortality among infected people and has a detrimental influence on a variety of clinical outcomes, including those of patients receiving cancer treatment, transplant surgery, or intensive care unit care [2]. MDR bacteria such as methicillin-resistant Staphylococcus aureus (MRSA), vancomycin-resistant Enterococcus (VRE), multi-drug-resistant Mycobacterium tuberculosis (MDR-TE), and carbapenem-resistant Enterobacteriaceae (CRE) are considered serious public health problem by WHO which calls for immediate action to mitigate their threats [3]. A form in which bacteria develop resistance to antibiotics and other environmental stress is through the formation of biofilm. Bacterial biofilms are organized communities of bacterial cells attached and embedded in a self-formed extracellular polysaccharide matrix (EPS) [4]. The assembled microorganisms present in biofilms may consist of different or the same microbial species and typically grow on organic or inorganic surfaces that serve as a source of nutrients [5]. Biofilm formation by microorganisms confers advantages such as protection from adverse environmental conditions (desiccation and starvation), host immune defense systems, and antimicrobial substances [6].
These advantages make biofilm a serious public health problem, as seen in the difficulties in treating biofilm-associated infections. The health implication of biofilm can be seen in the data presented by the Center for disease control in 2007, which state that “in the USA, about 1.8 million nosocomial infections were biofilm-associated, leading to a severe economic loss of over $11 billion” [6]. Furthermore, biofilm-related infections are responsible for more than 500 deaths annually, with a high treatment cost of over $94 billion [7]. The majority of persistent infections in humans, such as chronic sinusitis, chronic otitis media, cystic fibrosis, valve endocarditis, and implant devices (urinary catheters, prosthetic joints, and heart valves), are biofilm-related [8,9].
Thus, treatment of biofilm-related infection is problematic due to the possession of structural dynamic properties such as EPS that limit diffusion of antimicrobial agents, rendering them inactive [1]. Biofilm eradication strategies involve the development of biofilm-inhibitory agents that prevent biofilm formation during the early stage and biofilm dispersal agents that interfere with biofilm cell assemblage. Antibiotics have been used to treat biofilms. However, biofilms are highly resistant to antibiotics [10]. Some anti-biofilm agents, such as antimicrobial peptides, quaternary ammonium compounds, antimicrobial lipids, anticancer drugs (mitomycin), and nitric oxide-releasing antibiotics, have been used for biofilm eradication. However, various disadvantages such as inherent structures and complicated nature of antimicrobial peptides, inherent toxicity of quaternary ammonium compounds and anti-cancer drugs, difficulty in handling nitric oxide, the activity of phenazines/quinolines against only Gram-positive organisms, and likely hold of developing resistance to antimicrobial lipids due to their presence in diets hinder the use of these agents [11]. Consequently, it is imperative to seek a more effective biofilm dissolution therapy. Recent advances in the field of nanotechnology have resulted in the development of nanomaterials and devices that have found useful applications in medicine. Interestingly, dendrimers have shown to be an alternative to conventional therapeutic agents in biomedicine and could be used to combat infections caused by multi-drug resistant pathogens, including those producing biofilm [12]. Dendrimers possess mono-dispersed and well-defined structures widely studied and applied in various biomedical fields, such as drug delivery systems, magnetic resonance imaging contrast agents, antiviral, and antitumor and antibacterial agents [13]. The high interest in dendrimers is due to their special properties, such as multi-valency, mono-dispersity, biocompatibility, high water solubility, and chemical modularity for multi-functionalization [14,15]. Multi-valency provides dendrimers with many different functional groups capable of interacting negatively with bacterial cell membranes, disrupting membrane integrity [14,15]. In addition, the deformable and flexible branches of dendrimers assist in the simultaneous binding of ligands and multiple receptors on a cell surface, inducing a strong avidity binding via the multivalent binding effect [16]. The application of dendrimers in controlling infections caused by microorganisms and biofilm eradication has been studied with more emphasis on its versatility as an antimicrobial agent and its structural ability to penetrate biofilm matrix. This review highlights biofilm formation, dendrimer classifications, functionalities, and its application as an anti-biofilm agent. Also, the limitations of dendrimers as drug delivery systems and anti-biofilm agents are summarized. Toxicity and strategies to modify dendrimers to overcome the various challenges in their application in conventional antimicrobial treatments are highlighted.
Biofilm formation
Bacteria exhibit two survival states: planktonic and biofilm (sessile). Bacteria form biofilms through complex and irreversible steps involving chemical, physical, and biological processes (Figure 1). Biofilm formation involves a series of stages. First, single planktonic cells roam and adhere to a surface. The surface provides a good conditioning environment for cell adherence for biofilm formation initiation. These cells become enclosed in exopolymeric materials. Adherent cells secrete an extracellular polymeric substance (EPS) and become irreversibly attached to the surface, resulting in microbial cell aggregation and matrix formation. The biofilm begins to grow and mature by forming water channels, microcolonies and water channel systems while also becoming extensively layered. When the biofilm matures, after reaching maximum cell density, it is considered a three-dimensional community. Lastly, the mature biofilm releases microcolonies of cells from the main community, allowing them to move freely to new surfaces and disseminate the infection to new areas [11].
Attachment
The formation of a conditioning layer is the first stage in biofilm development. Fluid components settle onto the surface at this stage, thus forming a layer called substratum. Generally, rough surfaces and hydrophobic materials are more conducive than smooth and hydrophilic surfaces during biofilm formation [17]. These surfaces become inhabited by microorganisms, which cause them to produce a surface charge [18], which aids in the attraction and adhesion of microbial cells of opposing charges. The presence of glycocalyx, pili, and fimbriae on the surface makes the organisms cling to the surface more securely [19]. The preliminary stage is reversible but can be irreversible if adhesion is greater than repulsion.
Growth (formation of microcolonies)
The bacteria community starts to grow once the adhesion is strong enough to utilize the nutrients. At this point, biological processes control how other materials adhere to surfaces. This is the outcome of multiple genes that produce surface proteins like porins being expressed [20]. The polysaccharides used to create the EPS layer are transported through porins. The microbial cells interact as the biofilm develops through the secretion of autoinducer signals (AIs) [20].
About 100 billion bacterial cells can be contained in a well-developed biofilm per milliliter. Hence, microbial communication is crucial. The microbial cells are divided into a wide variety of communities, and each community is in charge of a specific task [21]. Another typical occurrence in a developing biofilm is the formation of tall, wrinkled structures, which exerts lateral pressure on cells by pushing the cells against each other. Dead cells in biofilms localize in the regions that encourage vertical bulging, which aids in relieving this pressure [22].
Matrix formation (metabolism)
The changes in the biofilm's environment result in changes in metabolic activity. The metabolic activity is high in the early stage of biofilm formation and gradually declines as development progresses [23]. Complex diffusion channels are utilized as the cell population grows for the circulation of nutrients, oxygen, and other components essential for cell growth. These channels are used to carry metabolic wastes and debris as well. The metabolic activities of the cells change in response to changes in the biofilm's environment. The gene expression in glycolysis is tightly regulated by conditions such as shear stress [24]. The bacteria that form biofilms tend to uptake foreign DNA, which could ultimately produce exogenous proteins [25]. Additionally, it was observed that some fatty acid-producing genes in some microorganisms are downregulated as biofilm develops [26].
Dispersion
Dispersion is the last stage, characterized by the shedding of biofilm, allowing the sessile cells to revert to their former motile forms. Lastly, biofilm spreads, colonizes, and establishes new locations using their inherent powers. Cell-cell communication is crucial for the pathogenicity and growth of biofilms. Quorum sensing is the communication primarily carried by autoinducers (AIs) (small diffusible molecules). AIs differ in various biofilms and depend on the type of microorganisms involved [27].
Composition of bacterial biofilm
Biofilms are made up of many components, such as the extra polymeric matrix (EPS) (which is the primary component), bacterial cells, secreted water, proteins, debris, and nucleic acid. These components make the free movement of materials and other important nutrients within the biofilm possible [28,29]. Biofilm arrangement is made primarily of two components: a region of tightly packed microbial cells with no obvious pores and a water channel for effective nutrient and other substance transport [30]. The configuration of microbial cells within biofilm determines the many physiological and physical characteristics of the biofilm.
Persister cells are also present in biofilm, rendering the human immune system and antibiotics ineffective. These are a few microbial cells that are resistant to antibiotic concentration and would normally be able to wipe out the majority of the bacterial population. The existence of these cells was discovered while researching the effect of penicillin on the streptococci population [31]. In addition to persisters, the biofilm activates various stress-related genes and factors that alter the resistant microbes to change properties such as temperature, pH, nutrition, osmolarity, and cell density, which alters the features of resistant microbes [32]. When circulatory systems and biofilm water channels were compared, their functioning was quite similar to that of early multicellular organisms [33].
Hydrodynamics and nutrient availability are two environmental elements that have an impact on biofilm formation and persistence. The biofilm is polymorphic, and studies with various glucose concentrations have shown that it can alter its form in response to the nutrients available in the environment. When the concentration of glucose rises, microbes quickly proliferate, and the thickness of the biofilm increases and vice versa [34]. Some studies have shown that different hydrodynamic conditions can alter the structure of biofilms. Bacterial microcolonies grow round in laminar flow, while in turbulent flow, they spread in the direction downstream [35].
Biofilms induce antibiotic resistance
Bacteria in biofilms are characteristically more tolerant to antimicrobial agents than planktonic cells of the same strain. While the antibiotic resistance mechanisms of planktonic bacteria are well known, those mechanisms (efflux pump, mutation, and antibiotic modifying enzymes) are not the main antibiotic-resistant mechanisms of biofilms [36].
The biggest obstacle after entering the biofilm is breaking through the compact matrix of the biofilm [36]. The drug's availability inside the biofilm is greatly decreased because the EPS absorbs it and prevents it from spreading throughout the matrix [37]. Additionally, EPS is characterized by tiny pores that can prevent bigger drug molecules from passing through [38]. Consequently, the antimicrobial treatment needed to get rid of biofilms maybe 1000 times more than needed for planktonic cells [39]. Also, inside a biofilm, antimicrobial agents that have penetrated may be enzymatically inactivated [35]. Inadequate oxygen and nutrient levels result in microbial cells being buried in the polymeric matrix, which makes them enter a stationary growth phase [40]. This phase reduces their susceptibility to antimicrobials that depend on the active growth of microbes [41]. In general, these factors make it extremely challenging to eliminate microbial cells deeply embedded in biofilms, resulting in recurring infections despite therapy (Figure 2) [42].
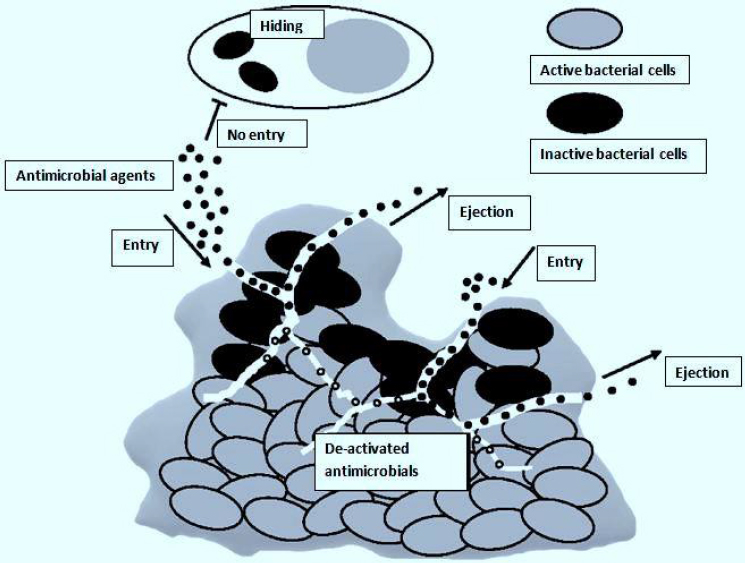
The effects of antimicrobials, once built up inside a biofilm, primarily depend on breaking down cell walls and possibly interfering with intracellular metabolic processes [42]. However, these won’t happen due to the effective defense mechanisms developed by biofilms, thus shortening the presence of antimicrobial agents [43,44]. Microorganisms develop resistance to many antimicrobial agents through horizontal antimicrobial resistance gene transfer, spontaneous mutation, activity of efflux pumps, and acquisition of exogenous resistance genes (Figure 2) [40,45]. Microorganisms such as Escherichia coli, Salmonella Typhimurium, Pseudomonas aeruginosa, and Candida albicans are only a few of the species whose ejection of antimicrobial agents from their cells is linked with the over-expression of efflux pumps [46-48]. Additionally, sub-inhibitory antimicrobial exposure level may lead to cell-wall thickening of bacteria due to mutation, acting as a barrier restricting antimicrobial agent penetration and their uptake by the microbial cells [49]. This was observed in erythromycin's decreased antibacterial activity against methicillin-resistant Staphylococcus aureus (MRSA) [50].
Due to changes in transcription and proliferation rates, biofilm cells have a considerably increased frequency of horizontal gene transfer, resulting in various phenotypes [51]. Furthermore, the distinctive structure of biofilms creates nutrients, ionic strength, pH, redox potential across the biofilm, oxygen generation gradient, a corresponding variation in metabolic activity, and biofilm growth rate at various strata [52].
Development of drug delivery systems
The eradication of infectious biofilms is a very difficult task. The use of traditional antimicrobial agents is complicated by numerous problems. To reach the target sites, antimicrobial agents must first "find their way" to a target without hurting the host. Naked antimicrobial agents without a drug delivery system frequently require a high dose to exert intended activities. This is because they lack target specificity and can harm the biological system [53]. Also, circulating antimicrobial agents can be deactivated by the immune system [54].
To activate a "stealth mode" and avoid the immuno-radar, an antimicrobial agent requires a pharmacological vehicle. Antimicrobial agents may not always be effective against resistant biofilm cells in laboratory experiments. If an adequate dose is used, increased antimicrobial resistance does not automatically indicate failure. The aforementioned difficulties would, however, be greatly exacerbated in a clinical setting because of a more complicated environment and the involvement of the host immune system [40]. To overcome these challenges, drug delivery methods that are target-oriented, biocompatible, non-immunogenic, and capable of penetrating biofilms and cell membranes must be created.
Potential prospects for eliminating pathogenic biofilms include developing a variety of site-specific targeting and effective penetrative drug delivery systems [55]. While antimicrobial resistance and recurrence of infections, as well as low pH settings, are shared characteristics of biofilms and tumors, they are also quite different from one other [56]. For instance, both of these have seen extensive applications of nanotechnology. Excellent biocompatibility, stability, and functionalization make nano-antimicrobial delivery systems target-oriented and environmentally sensitive [57].
In addition to the conventional chemical conjugation of antimicrobial agents to a carrier [58], a more appropriate method is to encapsulate antimicrobial agents into a vehicle that minimizes toxicity and unwanted side effects while protecting the cargo from degradation and deactivation [59]. These vehicles are also made to be exceptionally stable for parenteral injection, enabling the slow release of drugs at infection sites [60].
Dendrimers as vehicles of antimicrobial agents targeting infectious biofilms
Dendrimers are spherical, nanoscale particles that resemble a tree because their branches extend outward from a central point (Figure 3).
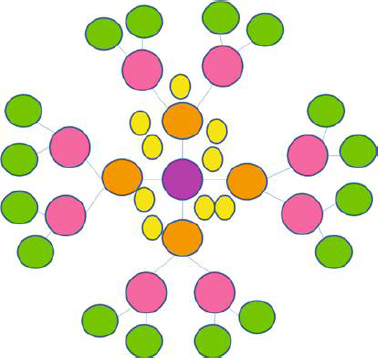
The pharmacokinetic profile of this macromolecule is greatly influenced by its structure, which is composed of a central core, repeating and highly branching subunits coupled to the former, and terminals attached with adjustable functional groups [62]. Its generation type (either half generation in the form of G0.5, G1.5, or full generation G1, G2, G3, G4, G5) is determined by the rise in branching in the form of radially homocentric layers, also known as dendrons, which is also indirectly responsible for the growth of its globular size. The loading capacity of dendrimers depends on generation, structure, and the peripheral conjugated functional group.
The capacity to encapsulate drugs concurrently rises with increased branching and internal core size [63]. Encapsulation, which entails enclosing the drug in the core hollow cavity, is one of the methods used for achieving drug delivery using dendrimers. Furthermore, covalent bonding with the surrounding functional groups can load antimicrobial agents onto the surface [13,64]. These techniques have long been used to synthesize various classes of dendrimers such as poly(amidoamine) (PAMAM), poly ether-copolyester (PEPE), liquid crystalline dendrimers, core-shell tecto dendrimer, poly(propylene imine) (PPI) dendrimers, glycodendrimers, polyglycerol, dimethylolpropionic acid dendrimers, chiral dendrimers, polyamido amine organosilicon dendrimers (PAMAMOS), poly(2,2-bis (hydroxymethyl) propionic acid dendrimers, and poly-L-lysine, poly(etherhydroxylamine) [62,65-71].
With its central core acting as the trunk and the branched dendrons arising from it, a dendrimer appears like a tree with many branches. The leaves of this branching, represented by the lateral functional groupings, are where it ends. The drug molecule is encapsulated by noncovalent interactions as a result of the interior cavity's click-in mechanism, which holds the drug moiety within the dendrimer and the number of branches surrounding the core cavity's shielding property from the outside environment.
When these weak hydrophobic and ionic bonds reach the optimal pH level, the amide group undergoes protonation, the host moiety separates, and the drug is released to the targeted cell [72]. Additionally, the charged functional groups on the surface of dendrimers exhibit effective electrostatic interaction with drug molecules of opposite charges. This kind of bonding improves the solubility of the drug. Drugs are conjugated with dendrimers via covalent bonds using specific chemical agents like polyethylene glycol (PEG), citric acid, macromolecules like saccharide [73], and para-aminobenzoic acid (PABA), which promotes increased stability, decreased toxicity, and controlled drug delivery [74,75].
Engineered dendrimers have a special ability for drug loading and delivery, and conjugating them with carbohydrates is fascinating for creating precise drug delivery systems. In addition to targeted delivery, dendrimers can be conjugated with carbohydrates to gain useful properties like bio-adhesion, stealth properties, solubility, biocompatibility, and decreased toxicity [63,76,77]. The therapeutic efficiency of antibiotics can be increased and adverse effects can be minimized by encapsulating them in dendrimeric systems. Controlling particle size, surface characteristics, functionality, branch length/density, and drug release are the main goals in the design of dendrimers as delivery systems to achieve the desired impact at the designated site of action [78]. The active molecules may condense inside the dendrimers, adhere to their surface chemically or physically, or both. These structures enhance the pharmacokinetic and pharmacodynamic characteristics of pharmaceuticals and can be used with more conventional drugs [79].
The PAMAM dendrimer is one of the most studied dendrimers for the release of antibacterial agents because of its hydrophilic characteristics, which are derived from many surface functional groups, making conjugation with antibacterial agents simple. The antibacterial capabilities can be improved when these dendrimers interact with water-soluble antibiotics. By substituting PEG or lauroyl chains for the amino-terminal groups of PAMAM dendrimers, it is possible to increase the substance's biocompatibility. Fluoroquinolones (nadifloxacin and prulifloxacin) antibacterial activity and water solubility significantly increased when conjugated with PAMAM G4 dendrimers containing ethylene-diamine surface groups (64 NH2 groups) [80,81]. To evaluate the resistance of Staphylococcus aureus and Cryptococcus pneumoniae strains to it, ciprofloxacin was loaded on basic PPI and PEGylated PPI dendritic structures. The antibacterial activity of the dendrimer loaded with ciprofloxacin was much higher than that of each of the individual components, showing that the conjugated system works in tandem [82].
Dendrimers
Dendrimers are globular hyperbranched nanopolymeric molecules with homogeneous, distinct, and monodisperse structures. They are made up of a central core moiety, repeating branching chains, peripheral reactive functional groups, and size ranges between 1 to 10 nm [83]. Dendrimers are classified based on their monomers, and their examples include polyamidoamine (PAMAM), polypropyleneimine (PPI), arborols, chiral dendrimers, and liquid crystalline, core-shell (tecto) [84].
The chemical and physiological properties of dendrimers depend on their monomers, generations (size), terminal groups, and synthetic routes. These parameters can be modified by dendrimers with specific properties for application in different biomedical fields [85]. While their characteristic properties vary, they have numerous characteristics that make them distinctive from other drug carriers. Explicitly, their biocompatibility, high degree of branching, water solubility and polyvalency make dendrimers an ideal carrier for various antimicrobial agents [86]. The numerous terminal functional groups of dendrimers, for instance, aid the conjugation of a wide range of biologically active moieties, such as chemotherapeutic agents, targeting agents, and nucleic acids. Dendrimers can also make it easier for numerous therapeutic agents to bind to their individual target molecules simultaneously by combining them into a single nanostructure [87]. This boosts the therapeutic efficacy.
Synthesis of dendrimers
Dendrimers are synthesized using two main methods: divergent and convergent methods. In the divergent method, the synthesis starts from the central core outward and presents (branches) functional groups for attachment or substitution with monomers. This method obtained the first generation (G1). This step is followed by the removal of inactive monomers, allowing for further binding of more monomers to ensure dendrimer growth [88]. In a study by Tomalia et al. [89], PAMAM dendrimer was synthesized using ammonia as the starting core; it underwent three Michael addition reactions with methyl acrylate. The terminal ester groups reacted with more ethylenediamine, forming a G1 dendrimer. Further amidation and Micheal additions resulted in newer generations [88].
Meanwhile, in the convergent method, synthesis starts from the monomers outside to the inside core [88]. The basic structure of the output molecule is predetermined and calculated by counting the branches connected to it. In this type of synthesis process, the new periphery molecule is activated for various reactions with monomers [90]. The convergent method is more advantageous because it has better structural control due to the low probability of side reactions, reduced amount of reagents, and production of pure compounds due to the purification process involved with each step [88]. However, the production of higher-generation dendrimers using the convergent method is challenging due to the steric effect observed in these macromolecules [91].
Classification of dendrimers
Based on property
Despite having a similar geometric architecture, dendrimers differ in terms of their physical and chemical characteristics.
Hydrophilic dendrimers: Generally, the most widely synthesized and commercialized are PAMAM dendrimers. Michael addition reaction is the first reaction that occurs in between an alkyl diamine core utilizing monomers of methyl acrylate to form a branched intermediate. The newly formed monomers, the reaction between ethanolamine and excess ethylenediamine, can be transformed into two smaller generation molecules, such as OH or -NH group surface group moieties, respectively [92].
This intermediate releases the smallest anionic dendrimers possessing four COOH groups on hydrolysis of the methyl group. When dendrimer development exceeds a certain threshold, the synthetic yield declines. The decline is due to the steric effect as a result of congestion of the branching arms (dense parking effect). Additionally, because of their increased water solubility, distinctive structure, and extensive range of surface groups, they are considered appropriate vehicles for the delivery of antimicrobial agents. They are available as methanol solutions and are obtainable commercially. The subclass of dendrimers with a tris-aminoethylene-imine core is known by the commercial name Starburst® dendrimers [92].
Biodegradable dendrimers: To create an ideal and huge molecular weight polymer with a high tissue deposition and quick clearance through urine to prevent nonspecific toxicity, biodegradable dendrimers were developed. In physiological solutions, they are frequently created by adding ester groups via enzymatic or chemical cleavage. The determining elements are the size of dendrimers, the monomeric unit’s lipophilicity, the type of chemical bonds, and the cleavage susceptibility of the internal and external dendrimer structures. Due to their biocompatibility and biodegradability, polyester dendrimers are used in gene therapy and anticancer treatments. However, the current study has switched to finding specific spatial and temporal degradation characteristics rather than the non-specific hydrolysis process and long-term deterioration characteristics [93].
Amino acid-based dendrimers: Blocks with various features, including hydrophobicity, optical property, chirality, and biorecognition, were integrated to create amino acid (AA) dendrimers. Generally, chirality in an atom is usually created by the joint action of the core and branching unit molecules with surface terminating groups. The unique internal structure created by amino acid building blocks offers stereoselective locations for non-covalently attaching molecules. Dendrimers are employed as targeted drug delivery systems, gene carriers, and protein mimics due to their distinctive structural folding of the unique branching units. These families of dendrimers are often created by grafting amino acids (AAs) or peptides into a regular dendrimer surface or by attaching AAs or peptides to an organic or peptide core (Figure 4a) [93].
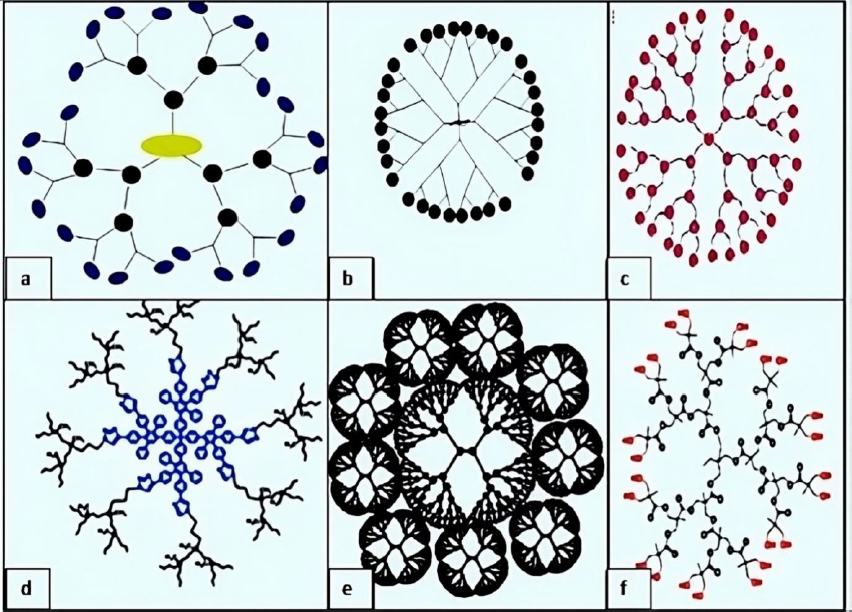
Glycodendrimers:The interaction of carbohydrates with numerous receptors on the cell surface, which in turn regulates several normal and aberrant processes, provides the basis for creating glycodendrimers. It was discovered that this interaction was potent for a multivalent ligand-receptor system. The findings from numerous studies led to the conclusion that carbohydrates were utilized as carriers in dendrimers. According to reports, glycodendrimers are used as a metastatic agent, an immune stimulant, and a carrier for cancer treatment (Figure 4b) [93].
Hydrophobic dendrimers: Water solubility must be high enough for dendrimers to be delivered systemically. However, the dendritic structure's hydrophobic void regions support the improved encapsulation and solubilization of lipophilic components. This structure resembles the micelle of an amphiphilic polymer, but it lacks the critical micellar concentration (CMC). Dendrimer building blocks are covalently bonded to one another, preventing them from disintegrating during the diluted solution phase. The solubility of hydrophobic dyes, probes, and fluorescent markers has been explored successfully in dendrimers, which have been found to exhibit hydrophobic interior gaps and hydrophilic surfaces mimicking unimolecular micelles.
Cyclophanes or dendrophanes are dendrimers reported to contain aliphatic and aromatic components. It has also been reported that these forms of dendritic structure regulate the release of drugs [93].
Asymmetric dendrimers: The bow tie polyester dendrimers (Figure 4f), which Gillies & Fréchet [94] formulated, are the most well-known asymmetrical dendrimers and may have a better pharmacokinetic profile. These are typically created by joining dendrons from different generations to a core linear molecule, forming a non-uniform orthogonal dendritic structure. In this form of dendrimer, the molecular weight, structure, and quantity of functional groups can be adjusted. Lee et al. [95] created a G3 asymmetric dendrimer via click chemistry.
Based on structure
Simple dendrimers: Simple monomeric units make up these kinds of dendrimers, which result from symmetrical substitution of benzene tricarboxylic acid ester. They contain 45 molecular diameters and 4, 10, 22, and 46 benzene rings connected symmetrically [96].
Crystalline dendrimers: Mesogenic monomers are used to form this kind of dendrimers by functionalizing carbosylane [97].
Chiral dendrimers: The chirality in these sorts of dendrimers depends on the construction of four constitutionally distinct but chemically related branches to a chiral core, such as chiral dendrimers made from pentaerythritol (Figure 4c) [98].
Micellar dendrimers: These dendrimers are water-soluble, completely aromatic, and hyperbranched polypropylene dendrimers that can produce a cluster of aromatic polymeric chains that can resemble some micellar structures and form complexes with tiny organic molecules in water [98].
Hybrid dendrimers: Peripheral amines in zero-generation polyethyleneimine undergo functionalization modifications, which results in the formation of these dendrimers. Columnar and cubic-like structures with a variety of structural characteristics arise as a result, and these structures experienced significant modification to give rise to dendritic structures such as hybrid dendritic linear polymers (Figure 4d) [99].
Amphiphilic dendrimers: They are prepared by segregation in which two sides of a chain are frequently split, with one side having electron-withdrawing capabilities and the other having electron-donating properties. These substances include, for instance, superfecta, hydraamphiphiles, and bolaamphiphiles [99].
Metallodendrimers: Metallodendrimers are formed using a complex method that can occur either inside the molecule or on its periphery. These dendrimers, such as ruthenium bipyridine, were discovered to have electrochemical and luminescent characteristics [96].
Tectodendrimers: Commercial tectodendrimers include Starburst® and Stratus® CS Acute CareTM. They have dendrimers in the center, and their functions range from identifying sick cells to determining the presence of infections (Figure 4e) [96].
Multilingual dendrimers: A multilingual dendrimer that is commercially available is VivaGel. On the surface, it contains several copies of a specific group of functions [98].
Multiple antigen peptide dendrimers: The poylysineskelton is used to generate a dendron-like structure in multiple antigen peptide (MAP) dendrimers. The amino acid lysine aids in conjugating the side chain of the alkylamine, which serves as a monomer for the different branching units. These dendrimers were formed and discovered to have a wide range of biological applications in diagnosis and vaccine production [100].
Drug encapsulation in dendrimers
The phenomena of drug release by dendrimers are dependent on the type of dendrimer and core moieties used. Different processes, including electrostatic encapsulation, covalent conjugation, and physical encapsulation, are used in drug release patterns [100].
Physical mode of encapsulation
By altering their shapes, cavities, and structural layouts, the molecules are entrapped in the inner moiety of the macromolecule using this technique. The internal cavities remain vacant, having two groups, lipophilic and hydrophobic interactions, which cause an interaction with the medicament molecules of nitrogen or oxygen atoms along with the release of the hydrogen bond. Several interactions, including physical and hydrogen bonding, led to the hydrogen bonding. Several drugs, including anticancer drugs like doxorubicin hydrochloride and methotrexate, can be encapsulated using this method [101].
Electrostatic interactions
Because dendrimers have several NH2 and COOH groups employed to increase the solubility of lipophilic drugs, the interaction in this type of encapsulation occurs on their surface. Easily ionizable drugs like ibuprofen, ketoprofen, diflunisal, naproxen, and indomethacin form complexes with multifunctional surfaces of dendrimers having terminal groups [101].
Covalent conjugation
This conjugation technique is employed when the compound has functional groups on its surfaces. In this technique, hydrophilic labile linkages are broken down chemically and enzymatically to form conjugated molecules. In addition, the stability and kinetics of the drug can be improved by using a spacer, as is the case with penicillin V, 5-aminosalicylic acid, venlafaxine, propranolol, and naproxen conjugated with PAMAM dendrimers. Other spacers include polyethylene glycol, aminobenzoic acid, lauryl chains, and p-amino hippuric acid. The consequence is increased solubility and regulated release of medications [102].
Applications of dendrimers in biofilm control
Highly branched three-dimensional structures called dendrimers have cavities that can be filled with both hydrophilic and hydrophobic substances [103]. Low-weight dendrimers have been reported to exhibit antimicrobial activity against S. aureus and E. coli without any loaded antibiotics [104] (Figure 5). Antibiofilm activities have been investigated in various studies. It was reported that the fucose-peptide dendrimer was highly effective in preventing P. aeruginosa biofilm formation by disrupting their membrane attachment [105].
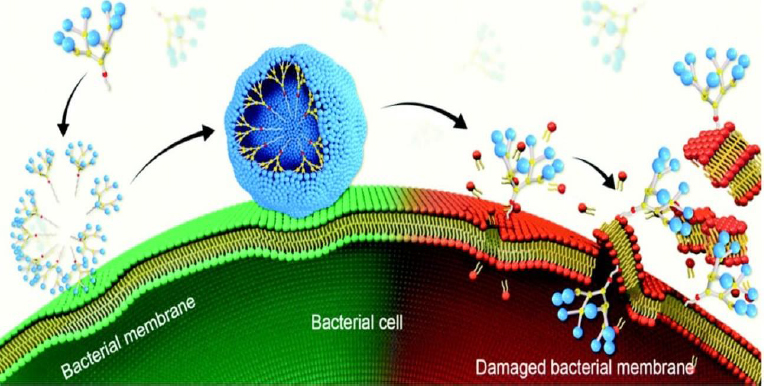
In another investigation, the antibiofilm activity of metallodendrimers comprising ruthenium(II) or copper(II) complexed with carbosilane dendrimer was evaluated. These metallodendrimers were found to inhibit S. Aureus biofilm formation and low hemolysis activity was observed [107]. Similarly, Han et al. [108] investigated the antibiofilm activity of lapidated TNS18 peptide dendrimer against P. aeruginosa biofilm. It was reported that TNS18 peptide dendrimer inhibited 90 % of biofilm formation, utilizing half of its minimum inhibitory concentration (MIC). P. aeruginosa's swarming motility was distorted at the same concentration by the dendrimer, and 55 % of the biofilms were dispersed at a 16-fold MIC concentration. Furthermore, Gide et al. [109] investigated the effectiveness of lipidated AMP-based dendrimers in preventing biofilm development. The dendrimer showed antibacterial action against both planktonic and biofilm cells and gram-negative and gram-positive bacteria, including MDR strains. By creating lysine branches, the researchers optimized the length of the fatty acid chain. The most effective substance, D-A-2, had a minimal hemolytic effect while being able to damage the membranes of MRSA and E. coli. Additionally, D-A-2 was able to completely halt the growth of E. coli and MRSA biofilms at a dosage of 0.8 μg/mL.
In another study, researchers investigated the role that various auxiliary groups played in dendrimer penetration of P. aeruginosa biofilms. Dendrimers with NH3+ groups at their perimeter penetrate P. aeruginosa biofilms' acidic environment more quickly than dendrimers with OH or COO groups. Electrostatic forces also led the peripherally charged dendrimers with NH3+ groups to be drawn to the negatively charged components of the biofilm, leading to aggregation around the top of the biofilm. Dendrimers possessing peripheral groups (OH and COO-) accumulate more frequently and are evenly distributed when they penetrate biofilms than NH3+ dendrimers. This means that the surface makeup of dendrimers can precisely control how well they penetrate and accumulate in biofilms, which is a crucial discovery for the ongoing development of novel antibacterial or antimicrobial-carrying polymers [110].
A study investigated the anti-biofilm activity of polyamidoamine dendrimers and polyaminophenolic ligands against mono- and multi-species legionella biofilms formed by L. pneumophila in conjunction with other bacteria prevalent in tap water, such as Escherichia coli, Aeromonas hydrophila, Klebsiella pneumonia and Pseudomonas aeruginosa. Cytotoxicity assay was carried out to test the concentrations of chemicals used as antibiofilm agents. The highest non-cytotoxic chemical concentration was used for biofilm inhibition activity, with dendrimer concentration tenfold being 10 times greater than polyaminophenolic ligands. Among the polyaminophenolic ligands chemicals, macrophen and double macrophen were the most active. Dendrimers were twofold more effective when compared with other chemicals, with a reduction of up to 73 and 85 % of multi-species biofilm and Legionella, respectively. These findings imply that the investigated compounds, particularly dendrimers, could be considered novel molecules in planning studies to create effective anti-biofilm disinfection methods for water systems to reduce legionellosis outbreaks [111].
The galactose-specific lectin LecA partly mediates the formation of antibiotic-resistant biofilms by P. aeruginosa, an opportunistic pathogen causing severe respiratory infections in cystic fibrosis and immunocompromised patients, suggesting that preventing LecA binding to natural saccharides might provide new opportunities for treatmentIn a study, convergent chloroacetyl thioether (ClAc) ligation between digalactosylated dendritic arms and 8-fold or 4-fold chloroacetylated dendrimer cores resulted in the formation of P. Aeruginosa biofilm inhibitor and 8-fold (G3) and 16-fold (G4) galactosylated analogs of GalAG2, a tetravalent G2 glycopeptide dendrimer LecA ligand. Biofilm inhibition assays, hemagglutination inhibition, calorimetry, and isothermal titration revealed that G3 dendrimers bind LecA slightly more effectively than their parent G2 dendrimers and cause complete biofilm inhibition and P. aeruginosa biofilm dispersal, whereas G4 dendrimers exhibit reduced binding and no biofilm inhibition. Based on the crystal structure of G3 dendrimer LecA complex, a binding model is formed to explain the observed saturation of glycopeptide dendrimer galactosyl groups and LecA binding sites [112].
A contributing factor in the development of antibiotic resistance in the opportunistic bacteria Pseudomonas aeruginosa is the establishment of biofilm, which prevents drug penetration. Hence, research focused on modifying tetravalent glycopeptide dendrimer ligands of P. aeruginosa lectins LecA or LecB to increase their antibiofilm activity. First, heteroglycoclusters were examined, showing one pair each of LecA-specific galacosyl groups and LecB-specific fucosyl groups and then binding simultaneously to both lectins, one of which provided the first fully resolved crystal structure of a peptide dendrimer as LecB complex, providing a structural model for dendrimer-lectin interactions (PDB 5D2A). By adding more cationic residues to these dendrimers, biofilm inhibition was increased, but bactericidal effects were equivalent to those of non-glycosylated polycationic antimicrobial peptide dendrimers. Another strategy involves creating dendrimers with four copies of Lewisa (a natural LecB ligand), which resulted in biofilm inhibition and slightly stronger LecB binding. Finally, excellent biofilm inhibition and dispersal were achieved by combining the antibiotic tobramycin with a LecB-specific nonbactericidal antibiofilm dendrimer at sub-inhibitory concentrations of both substances [113].
To probe if LecB inhibition affects these processes, high-affinity ligands were obtained by screening two 15,536-member combinatorial libraries of multivalent fucosyl-peptide dendrimers. The most effective LecB ligands observed were dendrimers PA8 (OFuc-LysAlaAsp)4(LysSerGlyAla)2 LysHisIleNH2 (IC50 = 0.11 mM by ELLA) and FD2 (C-Fuc-LysProLeu)4(LysPheLysIle)2 LysHisIleNH2 (IC50 = 0.14 mM by ELLA). Dendrimer FD2 activity resulted in the complete inhibition of P. aeruginosa biofilm development (IC50 10mM) and also led to the complete dispersal of already established biofilms in several clinical and wild-type strains of P. aeruginosa isolates. These studies imply that LecB inhibition by highly-affinity multivalent ligands can be a therapeutic strategy for treating P. aeruginosa infections by preventing the development of new biofilms and the spread of existing ones [114].
G3KL and TNS18 peptide dendrimers prevent the growth of Pseudomonas aeruginosa biofilms below their MIC value by affecting their swarming motility, as reported by Han et al. [107]. A higher concentration above the MIC was, however, needed to eradicate the preformed biofilm. Following observation using a scanning electron microscope and confocal laser micrographs, peptide dendrimers were shown to destroy biofilm morphological structure completely in a dose-dependent fashion. Long hydrophobic alkyl chains with tiny hydrophilic poly(amidoamine) dendrons with different terminal functionalities make up amphiphilic dendrimers. Astonishingly, the amphiphilic dendrimer containing amine terminals demonstrated strong antibacterial action against both Gram-positive and Gram-negative bacteria as well as drug-resistant bacteria, and it inhibited the formation of biofilms [106].
Advantages and limitations of dendrimers as a drug carrier
Dendrimers are hyperbranched polymers created in layers around a central core [115]. These structures are, therefore, very reactive to bacteria in vivo due to their larger surface area to size ratio and hydrophilic structure. Additionally, these structures possess a high density of functional groups attached for enhanced targeted delivery [115].
The properties of dendrimers, including chemical reactivity, solubility, and glass transition temperature, depend on the nature of the end group. The solubility of dendrimers varies with the diversity of functional groups. When hydrophilic groups are present, they are highly soluble in polar solvents, and when hydrophobic groups are present, they are soluble in nonpolar solvents [116]. Their higher generation results in an increase in cubic volume. Dendrimers have a great degree of structural control and are typically compact, spherical, or globular in shape. They have high ionic conductivity, reactivity, water, and non-polar solubility.
Dendrimers are a great choice for target bio-imaging and diagnostics because of their ability to adjust their properties based on their shape, solubility, mono-dispersity, and the simplicity with which huge doses of substances can be loaded [117]. To improve the selectivity and bioavailability of hydrophobic drugs at the point where they bind to biofilms, several approaches are being used. Also, clearance through the reticuloendothelial system is considerably reduced due to its size [62]. Dendrimers feature surface functional groups that can act as vectors to target a particular spot and deliver drugs specifically to biofilms.
Due to their structural specificity, dendrimers make ideal partners for active pharmaceutical ingredients, which allows the following: inclusion of pharmaceutical compounds inside the cavities (Figure 6a), attachment of pharmaceutical compounds to the functional groups at the periphery of the dendrimer (Figure 6b), and both offering encapsulation (internal cavities) and support for conjugates (on the surface) (Figure 6c).
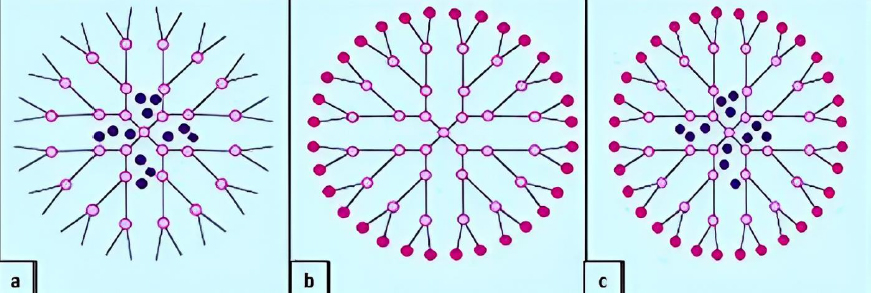
The interaction between pharmaceuticals and dendrimers is advantageous because it increases solubility, which enhances the drug's toxicity, absorption, and bioavailability [118].
Dendrimers provide several advantages over other polymeric architectural systems. Dendrimers' branched macromolecule properties are entirely distinct from modern linear polymeric materials [83]. Such variations are frequently caused by the method of synthesis. While the methods for developing dendrimers can produce homogeneous structures with uniform molecular weights, conventional polymerization typically results in polydisperse structures with various molecular weights [120]. Dendrimers are referred to as "host-guest" molecules because of their spherical shape and internal chambers, which allow them to demonstrate excellent encapsulation properties and convey many substances in their interior. Two or more molecules/ions are held together in host-guest systems by unique structural connections. They are interconnected by forces other than fully covalent bonds [121].
The high solubility and permeability of dendrimers have reportedly been attributed to the presence of many surface functional groups. The terminal groups can be very reactive at times, necessitating additional changes. It is possible to change a macromolecule's physicochemical properties or create a specific function, such as a catalytic or medicinal one, by post-modifying its surface [122].
The dendritic architecture and several secondary amine groups in the structure of the dendrimers provide more drug-loading reaction sites. Engineered dendrimers have a special ability for drug loading and delivery, and conjugating them with carbohydrates is fascinating for creating precise drug delivery systems. In addition to targeted delivery, dendrimers can be conjugated with carbohydrates to gain useful properties like bioadhesion, stealth properties, solubility, biocompatibility, and decreased toxicity [77].
Despite these unique features, their usage is restricted because of their rapid in vivo clearance and significant cytotoxicity under physiological settings [123,124]. Regardless of dendrimers' advantages as drug delivery vehicles, there are still certain problems to be resolved. Dendrimer toxicity and biodistribution are tightly correlated with their size and surface chemistry [122]. The main issue is a size restriction. Generation 6 and higher PAMAM dendrimers require a greater reliance on the hepatic clearance pathway for clearance, while generation 5 or lower dendrimers can effectively be removed via glomerular filtration and the renal excretion pathway [125]. Dendrimers with diameters ranging from 4 to 10 nm can cross the cellular endocytosis barrier and interact with nanometric cellular components [122]. However, generation 6 and higher PAMAM dendrimers are quite expensive and poisonous [64]. As a result, PAMAM dendrimers from higher generations are rarely employed. Cationic dendrimers have a strong ability to bind to nuclei or anion molecules, which help to internalize cells [62]. However, non-specific plasma protein adsorptions and reticuloendothelial system-accelerated elimination are frequent problems for cationic dendrimers [122]. Additionally, there is a limited intracellular dissociation of dendrimers with nucleic acids [126]. Cationic dendrimers are more hazardous than neutral or anionic dendrimers, especially at high dosages, since their interaction with cell membranes (negatively charged) can promote cell membrane instability, leading to cell lysis [127,128].
In research where dendrimers were tested in already-existing biofilms, disruption was not seen despite the encouraging results against planktonic bacteria. The researchers speculated that the dendrimers' huge size, which prevents their entry into the biofilm's matrix and limits them to the surface of the biofilm, may be the cause of their ineffectiveness against mature biofilms [129]. Additionally, the dendrimers demonstrated toxicity in cytotoxic tests utilizing the A549 human lung cancer cell line and hemolysis assays at concentrations within the same order of magnitude as the MIC values determined for the examined bacterial strains [129]. According to the authors, this data shows that dendrimers have a wide range of activities on both bacterial and human cells. These dendrimers have antibacterial action, but their antibiofilm activity needs to be enhanced with reduced cytotoxicity for therapeutic applications. Therefore, the efficiency of dendrimers is influenced by the length of the alkyl chain, dendrimer synthesis, and bacterial strain [130,131].
Cytotoxicity of dendrimers
As a potential therapeutic agent, dendrimers have shown significant contributions in the biomedical field. However, just like any other therapeutic agent, toxicity study is of great importance to assess its safety for biological applications. As highlighted earlier, the toxicity of these nano-carriers is related to their size and structure. Dendrimers are nanosized, enabling them to interact with many other cellular components, including nucleic acids, proteins, plasma membranes, ions, heavy metals, vitamins, and organelles (mitochondria, endosomes, nuclei) [62].
In general, the toxicity of dendrimers can be related to their physicochemical properties, such as size, generation, charge, and concentration. The cytotoxicity of dendrimers is dependent on size and generation. The cytotoxicity of low-cationic generation is normally lower than that of high-generation dendrimers due to the increased number of positive charges associated with them [132]. It was reported by Han et al. [133] that PAMAM G3 caused 80 % hemolysis after 24 h, G4-NH2, and G5-NH2 dendrimers caused 100 % hemolysis within 4 h, while PAMAM G6-NH2 dendrimers call caused total hemolysis in 2h.
It has been shown that the toxicity of dendrimers is highly dependent on surface charge (anion, cation, and neutral). Cationic dendrimers exhibit a high level of cytotoxicity when compared to anionic dendrimers [134]. An in vivo study using an embryonic zebrafish model showed that surface greatly influences the toxicity of dendrimers [128]. Positively charged dendrimer cores showed considerable cytotoxicity and drastic phenotypic alterations when dendrimers were used as nanocarriers, in contrast to negatively charged dendrimer cores [135]. The maximum tolerance value (MTV) can be used to express the toxic concentrations of cations; a value above the MTV demonstrates concentration-dependent toxicity, whereas anions and neutrals hardly exhibit cytotoxicity over a wide range of concentrations (except for extremely high concentrations), both in vivo and in vitro. In an in vivo test, the MTV cytotoxicity of cationic dendrimers (PAMAM) in the mouse stomach ranged from 30-200 mg/kg, while the MTV of anionic dendrimers reached as high as 500 mg/kg (below G7) [136]. Naturally, hemolytic concentration is another logical indication of toxicity evaluation in addition to MTV. High-production cation dendrimers demonstrate the highest levels of hemolytic toxicity and cytotoxicity (at 90 nM, G6). High-generation cations have substantial toxicity at low concentrations. However, they do not exhibit toxicity below a particular level (below 0.009 nM, G6) [133].
There are different ways of evaluating the toxicity of therapeutic agents, either by assessing their activity against microbial cells, body cells, or tissues. This review focused more on the cytotoxicity of dendrimers on the body cells and tissues. By staying in circulation for extended periods and staying in contact with blood flow components, dendrimers enhance sustained systemic distribution [137]. However, when cationic dendrimers enter the blood, they interact with the proteins and cells (RBCs, white blood cells, and platelets) to cause hemolytic toxicity and alter hematological parameters.
Cationic PAMAM dendrimers G4, G5, and G6 were demonstrated to cause the aggregation of human platelets, as measured by particle size and surface charge analyses, rather than by altering the integrity of the plasma membranes of platelets [138]. Dendrimer nanocarriers prevent immunotoxicity but can also have the opposite effect on the body [139]. By using immunoprecipitation and Ouchterlony double-diffusion experiments, Roberts et al. [140] attempted to determine the immunogenicity of cationic PAMAM dendrimers G3, G5, and G7 with an amino surface in rabbits. The blood-brain barrier (BBB) prevented the majority of drug delivery methods from accessing the brain, making drug delivery in the central nervous system difficult. A significant issue is how harmful drug delivery systems are to the central nervous system [141]. Dendrimers can carry drugs or genes to treat brain or nervous system diseases over the BBB, but it is important to consider their potential for neurotoxicity [142]. Similar to dendrimers, dendrimers' structural features have been shown to cause a variety of neurotoxicological effects in living things. Following oral administration, PAMAM dendrimers can be physiologically distributed in the heart, lungs, liver, blood, urine, stomach, and small and large intestine [143]. Tissue accumulation of Organs like potential reactive oxygen species (ROS) producing dendrimers, wide distribution of phagocytes, and particularly, the slow clearance of dendrimers renders organs like the spleen and liver the main target of dendrimers toxicity. In addition, dendrimer exposure may impact high-blood flow organs, including the kidneys and lungs [144]. Few researchers, however, have looked at the organ-specific toxicity of dendrimers. More thorough toxicity studies on each organ are required for the safety of administration [145]. Specific toxicity assay of organs will be vital to control the dose and reduce potential side effects. The presence of autophagic vesicles in hepatocytes, hepatocyte necrosis, and vacuolation was seen in mouse liver sections after exposure to 100 g/mL of PAMAM dendrimer G5 for 24 h. When mice were given dendrimers, the administration of the autophagy inhibitors 3-methyladenine (3-MA) and chloroquine (CQ) led to the recovery of liver weight loss, a reduction in liver tissue damage, and the blocking of serum biochemical parameters, which suggests that PAMAM dendrimers may damage liver tissue through autophagy [146]. Dendrimers can penetrate the digestive tract after oral administration and cause harmful effects on the gastrointestinal epithelium [147]. In isolated rat jejunum displayed in chambers, PAMAM dendrimers were demonstrated to penetrate the intestinal epithelium. However, 1 mM G3.5 and G4 PAMAM dendrimers failed to promote paracellular transport to isolated rat tissue and harmed rat jejunal cells. This discrepancy might have occurred because the supporting cells and mucus beneath the epithelial barrier of the rat jejunal mucosa were less susceptible to permeability than the Caco-2 cell culture [148].
Following local administration of PAMAMNH2 G2 and G3 dendrimers (at a dosage of 6 mg/mL), skin irritation tests on rats demonstrated minor erythema and evident alterations in the shape of epidermal cells. Rats treated with PAMAM-NH2 at much higher doses (30 or 300 mg/mL) exhibited moderate to severe erythema as well as clear histological alterations in the dermis. Additionally, when exposed to high doses of PAMAM-NH2 dendrimers, PCNA was strongly expressed in all skin layers and the nuclear immune response was boosted, suggesting cell proliferation disorder. Immunohistochemical studies and microscopic evaluations revealed that cationic PAMAM dendrimers quickly caused significant skin toxicity and that low concentrations should be considered for real-world external application [149].
Dendrimers modification
Dendrimers can be used as the foundation for bigger nanoparticles through the development of certain strategies. For instance, dendrimer clusters can create size-switchable or adaptable nanoparticles [149]. Most of them are 100 nm in size, and they don't move around while blood circulates. When nanoparticles reach the desired tissues, they break apart and precisely release individual dendrimers, allowing them to use their exceptional tissue penetration ability and cell internalization properties. Goa et al. [150] created charge and size adaptive clustered nanoparticles based on the electrostatic interaction between PAMAM and 2,3-dimethyl maleic anhydride modified poly(ethylene glycol)-block-polylysine (PEG-b-PLys) [150]. The outside layer of the clustered nanoparticle is made up of PEG chains, and the inner core is made up of complexes of PAMAM with PLy chains. The clustered nanoparticles were larger than 100 nm and contained peripheral PEG chains. They were shown to have prolonged blood circulation and a negatively charged surface of about -2.2 mV. The dendrimer and PEG-b-PLys were broken down when they reached the sick lung tissue because the acidic microenvironment caused the carboxyl groups in the PLy segments to change to amine groups. The released PAMAM dendrimers successfully penetrated and were retained inside biofilms by utilizing their tiny size (6.5 nm) and positive charge (23.8 mV). Charge-reversal dendrimers for surface stealth modification during body circulation, PEG is a non-immunogenic, inert, non-antigenic polymer with great biocompatibility and water solubility. It has been approved by FDA [151].
Dendrimers are frequently PEGylated to protect their cationic surfaces, lessen their toxicity, and extend their circulation times [152]. By lowering unspecific absorption, PEGylated PAMAM dendrimers lengthen systemic circulation duration and boost the concentration in the target tissues [153].
Deactivating the cationic surface charges of dendrimers is another method to lessen non-specific cellular uptake and adsorption during blood circulation. The positive surface charges can then be activated inside the target tissues or cells. Based on this idea, charge-reversal or charge-switchable dendrimers can be created. Parameters such as temperature, osmotic pressure, pH, and biological signals may be employed to reverse charges on the dendrimer surface [56,154].
Another method of reducing the toxicity of dendrimers is through acetylation. When the acetyl group is conjugated with the terminal group, the positive charges on the surface of the dendrimer are neutralized. It was observed that acetylated dendrimers exhibit higher solubility, which is a special property for drug development and biological applications [119]. In addition, attention needs to be given to anionic or half-generation dendrimers. This is because previous research has shown that the toxicity of dendrimers is caused by the presence of polycationic charges on their surface, and full-generation dendrimers are characterized by the presence of polycationic functional groups, while half-generation contains the carboxylic acid functional group. Hence, research should be focused on half-generation to improve its biocompatibility. Also, dendrimers with negative charges on their surface were shown to exhibit negligible toxicity and hemolytic effects [155].
Conclusions
Bacterial pathogens have developed many mechanisms to resist antimicrobial agents, and the formation of biofilm remains one of their most effective resistance mechanisms. Biofilm formation by pathogens protects the organisms against antimicrobial agents and from adverse environmental conditions (desiccation and starvation) and the host immune defense systems. Hence, biofilm-associated microbial infection constitutes a serious public health problem. Recent advances in nanotechnology and the fabrication of various nanomaterials and dendrimers have shown enormous promise. Dendrimers showed a tremendous ability to inhibit biofilm formation and development due to their special properties such as -dispersity, biocompatibility, high water solubility, and chemical modularity for multi-functionalization. Also, due to their unique structural organization, drugs can be incorporated into dendrimers through molecular modifications. However, just like other antimicrobial and antibiofilm agents, dendrimers showed moderate cytotoxicity related to their size, generation, charge, and concentration. Despite dendrimers' advantages as drug delivery vehicles, there are still certain problems to be resolved. Dendrimer toxicity and biodistribution are tightly correlated with their size and surface chemistry. Modification of these parameters can help shape dendrimers to be effective antibiofilm and drug-delivery agents to curtail the menace of biofilm-related infections. Advancement in computational modeling and analytical techniques is necessary for future research because it will provide a deeper understanding of dendrimer properties and activity, accelerating the development of new dendrimers with improved features. This can be tailored to design potent and selective dendrimers that can effectively target and disrupt biofilms with less cytotoxicity.