INTRODUCTION
Pediatric patients often require individual dosing of medications, even in cases where standard doses for adults are available. Pediatric patients exhibit altered pharmacokinetics (PK) compared to adults, leading to a need for dose calculations based on factors such as a child's age, weight, and body surface area (1). During a child’s growth, the developmental changes in metabolic capacity, distribution sites, gastrointestinal function, acquisition of the renal function, and integumentary development occur rapidly and determine age-related dose personalization (2). Non-individualized pediatric medications present a tolerability risk as a result of overdosing or the lack of efficacy by underdosing.
To address palatability, dosing accuracy, and swallowability concerns with regard to pediatric patients, multiparticulate oral solid dosage forms have been introduced (3). However, manually compounded liquid dosage forms (suspensions, emulsions, solutions) still present a well-established widely used approach for flexible dosing in oral drug delivery (1). Nevertheless, extemporaneous preparations come with some setbacks. The palatability of the liquid dosage is a major issue, especially when the taste sensation difference between individuals is considered. In addition, taste perception varies between cultural environments, making it difficult to find globally acceptable flavors (4). Dosage precision is another issue with liquid dosage forms, such as reconstitution errors carried out by inexperienced caregivers, with several studies indicating suboptimal dosing accuracy with spoons and cups (5). For instance, under-dosing of antibiotics might lead to the risk of microbial resistance, and over-dosing might result in toxicity (6). Tablet crushing or extemporaneous modifications of marketed medications often lack dose accuracy and might change the bioavailability, toxicity, and stability of the drug product (7). For instance, mixing crushed tablets with liquid vehicles or food might alter the pharmacokinetics of the active pharmaceutical ingredient (API) (e.g. thickening agents could hinder drug release) (8).
An automated manufacturing approach such as 3D printing could circumvent the mentioned setbacks and address the need for individualized dosage forms. This paper discusses how 3D printing can address the challenges associated with pediatric medication and provide personalized doses of medication for each individual patient. In addition, various aspects related to technology, quality, manufacturing, intellectual property, and regulation will be highlighted.
TECHNOLOGICAL OVERVIEW OF 3D PRINTING
3D printing or additive manufacturing is an umbrella term for a variety of technologies, where the objects are produced in a layer-by-layer process based on digital models (9). Numerous 3D printing technologies with different raw materials (gels, pastes, polymers, powders, liquids, etc.) and processing principles can be utilized (10, 11). Common denominators for most 3D printing processes are summarized below (Fig. 1) (12):
Digital design. The process starts by designing an object using computer-aided design (CAD) software.
Conversion of the design to 3D printer-compatible format. CAD file is converted into printable layers by slicing of external surface. The printing instructions are transferred to the 3D printer. Multiple settings (speed, temperature, infill pattern, etc.) are defined at this step and might have a critical influence on the product.
Raw material preparation. To facilitate the printing process, raw materials may be processed into pastes, gels, granules, filaments, or binder solutions.
3D printing. Materials are added and solidified in an automatic layer-by-layer process to produce the final object.
Post-processing. 3D printed products may require drying, curing, sintering, or excess powder removal after the printing process is completed. In some cases, the excess material may be collected and recycled for the next printing process.
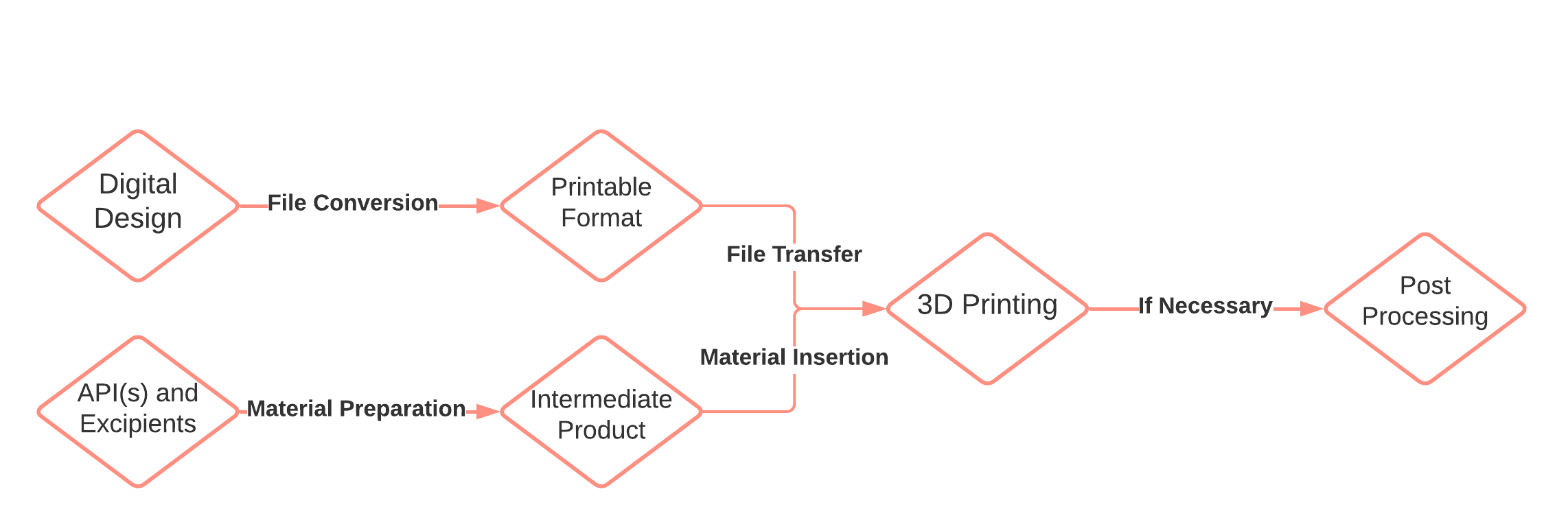
Fig. 1. Flow chart of the 3D printing process.
Several 3D printing technologies have already been evaluated for pharmaceutical applications, such as stereolithography, selective laser sintering, inkjet printing, binder jetting, fused deposition modeling and semi-solids extrusion (13–15) (Table I). In general, the above-mentioned printing approaches can be categorized into extrusion-based, powder-based, and liquid-based operations based on working principles. There are also other 3D printing approaches with limited applicability for pharmaceutical applications.
Table I. Summary of main pharmaceutically applicable 3D-printing technologies
The foundation of extrusion-based printing technologies is material deposition through a heated nozzle into a final dosage form (16). The most commonly explored printing technologies in this category are fused deposition modeling (FDM) and semi-solid extrusion (SSE). Based on the author’s opinion, both technologies have a high potential for personalized medication preparation at the point of care, as they offer a high degree of freedom in personalization options with regard to selected dosage, release kinetics, and tablet shape (17, 18). FDM is the most popular technology for the drug delivery system today (Fig. 2a) (14). A thermoplastic polymeric thread – the filament – is loaded into the heated print head via a feeding mechanism. The filament is an entry feedstock for the 3D printer and is prepared separately by hot melt extrusion (19). Once the filament is softened, thin strands are deposited through the nozzle onto the print bed, where the material cools and solidifies (20). Subsequently extruded layer is fused with the previously deposited layer to create a strong bond. No post-processing is required after the object is formed. Additional advantages of FDM include low equipment cost and good mechanical properties of the printed dosage forms (21). A crucial limitation of FDM is the use of high printing temperatures, usually above 120 °C, which may induce drug degradation (22, 23). To avoid the filament preparation step, FDM can be upgraded into a direct extrusion system, where powders or pellets are heated within the printhead and extruded directly into a final dosage form (24, 25). On the other hand, SSE is based on pneumatic or mechanical extrusion of gels or pastes through a syringe-like mechanism in a layer-by-layer fashion (Fig. 2b) (13). Syringes are prefilled with semi-solid material, which is prepared by mixing optimal ratios of API, polymers, and appropriate solvent(s) (26). SSE allows for room or slightly elevated processing temperatures, while a wide array of pharmaceutical-grade materials are available (12). Therefore, drug degradation due to thermal input is negligible (27). Nevertheless, comprehensive formulation studies are necessary to avoid low-resolution printing and post-printing deformations after the completed printing process (12, 28). In addition, a lengthy post-processing step is required to ensure complete solvent removal from the dosage form, as excessive solvent presence can lead to moisture-related drug degradation or toxicity (28, 29). Overall, FDM and SSE offer a potentially useful alternative to current compounding approaches.
The working principles of the powder-based printing category involve powder deposition in several fine layers, where particles are bound together by an external source after each deposition. In the case of selective laser sintering (SLS), a high-power laser beam melts and sinters the powder particles together in a compact layer (Fig. 2c) (30). After the first layer is completed, the printing process alternately continues between powder deposition and sintering, until the final dosage form is produced and subsequently recovered from the powder bed (14, 31, 32). A key benefit of SLS is the absence of any solvent and the potential for high-resolution printing. On the other hand, SLS involves exposure of materials to high temperatures and high-energy lasers, which could lead to drug degradation (33). An alternative technology to SLS is binder jetting, where the particles are bound together by a binding liquid instead of a high-energy laser (Fig. 2d). The binding liquid is sprayed on the surface of the powder bed after each powder deposition cycle (34). Liquid bridges are created between particles, which are solidified after drying in a process similar to granulation (35, 36). Binder jetting was used to manufacture the first FDA-approved 3D printed medication Spritam® by Aprecia Pharmaceuticals on a large scale. In addition, various pharmaceutical-grade excipients are compatible with the technology (37). The main limitation of binder jetting is a rather fragile character of the manufactured dosage forms, as tablets are prone to damage during handling and transport (14, 38). This is because of the high porosity of the printed dosage forms and the limited binding ability of the liquid. With suitable formulation and process approaches, the fragility can be mediated to an extent. Nevertheless, selective laser sintering and binder jetting have a lot of potential for pharmaceutical 3D printing. While there are arguably fewer personalization options with regards to tablet shape and release kinetics, scaling the production process is achievable. Both technologies produce dosage forms that rapidly orally disintegrate, typically within a few seconds (37, 39–41). This orodispersible feature can aid in administration and swallowability for pediatric patients. An important limitation is that both technologies leave residual powder around the powder bed and on the surface of the dosage form or manufacturing platform. However, the excess powder blend can be collected and re-used for future printing cycles, as long as powder blend segregation is avoided (42, 43). Extracting the tablets from the powder bed is a complex operation that demands delicate handling and specialized equipment to avoid contamination and ensure the integrity of the final product (32, 44). Therefore, SLS and binder jetting are primarily suitable for large-scale production environments rather than compounding pharmacy offices.
Lastly, liquid-based printing technologies include API-containing liquids, which are converted into the dosage form. This category might be less applicable to the pharmaceutical environment, even after being extensively researched. Stereolithography (SLA) is based on the curing process of photosensitive liquid resins ( e.g. polyacrylates and epoxy macromers) by a UV light, where the resin is solidified into a final dosage form (Fig. 2e) (13, 35). Unfortunately, SLA might introduce an element of toxicity to the dosage form due to the nature of the material. Uncured resins contain a high concentration of free radicals, while the long-term stability of photosensitive materials is also questionable (45, 46). Other limitations include the potential for interactions between polymers and APIs, lack of FDA-approved excipients, and a lengthy post-processing operation (46, 47). Inkjet printing is a liquid deposition technique that can be used to apply APIs, polymers, and other excipients onto an edible substrate (Fig. 2f) (48). The API solution is sprayed through a series of nozzles in a single or more layers. The dried liquid forms a thin film that disintegrates upon contact with saliva (49, 50). While this technology also offers the advantage of rapid disintegration, it is limited by the dosing possibilities and size of the printed object. Therefore, inkjet printing primarily enables the preparation of mostly low-dose solid dosage forms (51–53). Normally, dosage forms of up to a few milligrams can be manufactured (54). This poses a significant challenge, as higher doses are often necessary to achieve therapeutic efficacy, even for pediatric patients.
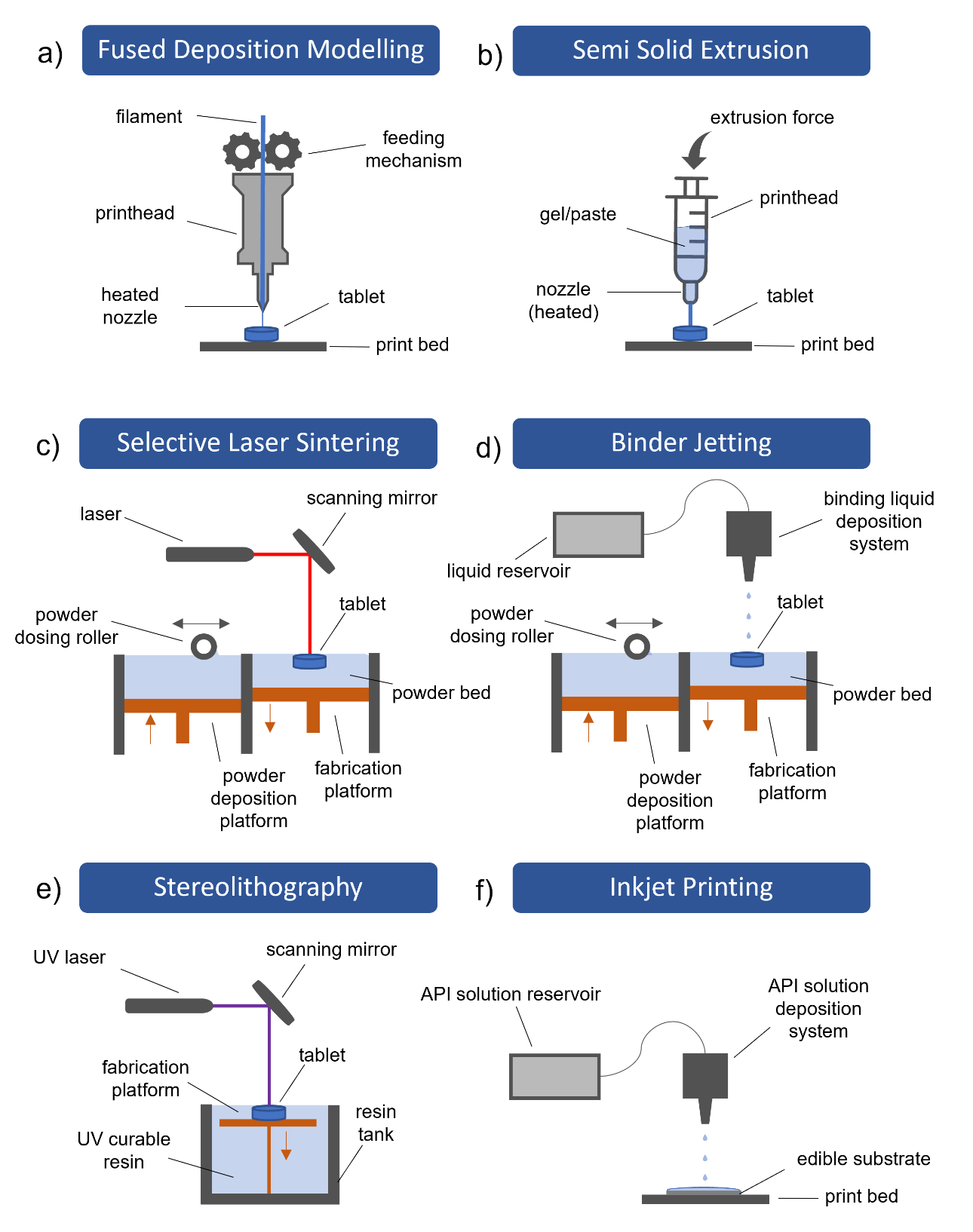
Fig. 2. Schematic overview of commonly used 3D printing technologies: a) fused deposition modeling, b) semi-solid extrusion, c) selective laser sintering, d) binder jetting, e) stereolithography, and f) inkjet printing.
All in all, a careful selection of suitable 3D-printing technology should be performed to develop a personalized dosage form. Extrusion-based 3D printing technologies have the potential for small-scale personalized medicine preparation at the point of care, while powder-based technologies might be more appropriate for large-scale manufacturing. Currently, there is no preferred 3D printing principle, as each includes key benefits and limitations.
PERSONALIZATION OPTIONS OF 3D PRINTED MEDICATIONS
With various options for personalization, 3D printed medications have the potential to improve drug delivery, meet specific patient needs, and enhance treatment outcomes. Prescribers can consider body weight, body mass index, and various biomarkers specific to each individual pediatric patient when preparing the personalized 3D printed medication (55). Physicians could adjust the treatment by taking into account caregiver’s and children’s preferences. In addition to dose personalization, 3D printing offers a versatile manufacturing platform that enables tailoring of tablet shape, palatability, release kinetics, and swallowability (Fig. 3) (56–59). This way, pediatric patients might better adhere to medication regimens. Opportunities for medicine personalization for pediatric patients primarily stem from the ability to select a custom size of dosage forms, chosen 3D printing technology, and tailored shapes.
The potential benefits of various 3D printing technologies for the pediatric population have already been extensively researched. Unique advantages related to swallowability and palatability have been attributed to some technologies. In a visual acceptability study, children chose chewable 3D printed tablets as a desired tablet formulation among other traditional solid tablets (65). A chewable character is a distinct advantage as pediatric patients are probably appealed to easier swallowing and familiar candy structures. Chewable dosage forms can be prepared with SSE by selecting appropriate excipients. 3D printed isoleucine chewable tablets were already prepared in a small experimental study with four children in Spain, which were comparable to manually compounded capsules based on isoleucine plasma concentration (66). Similarly, it has been demonstrated that children prefer orally disintegrating dosage forms (55). These can be manufactured by SLS and binder jetting. Orodispersible 3D printed formulations disintegrate instantly with a sip of water (40, 67). The first FDA-approved 3D printed medication Spritam® specifically includes the orodispersible feature to address the swallowing difficulty (68). Furthermore, immediate, delayed, pulsatile, and prolonged release profiles can be achieved by choosing fitting materials and technologies (69–72). Prolonged or delayed drug release would enable children to take their medication in a familiar setting in the morning or evening instead of relying on daycare (55). Completely new pharmacokinetic features of dosage forms can even be developed, such as the two-pulse release profiles or a multi-drug combination therapy ( e.g. polypill) with customized release profiles (73–75).
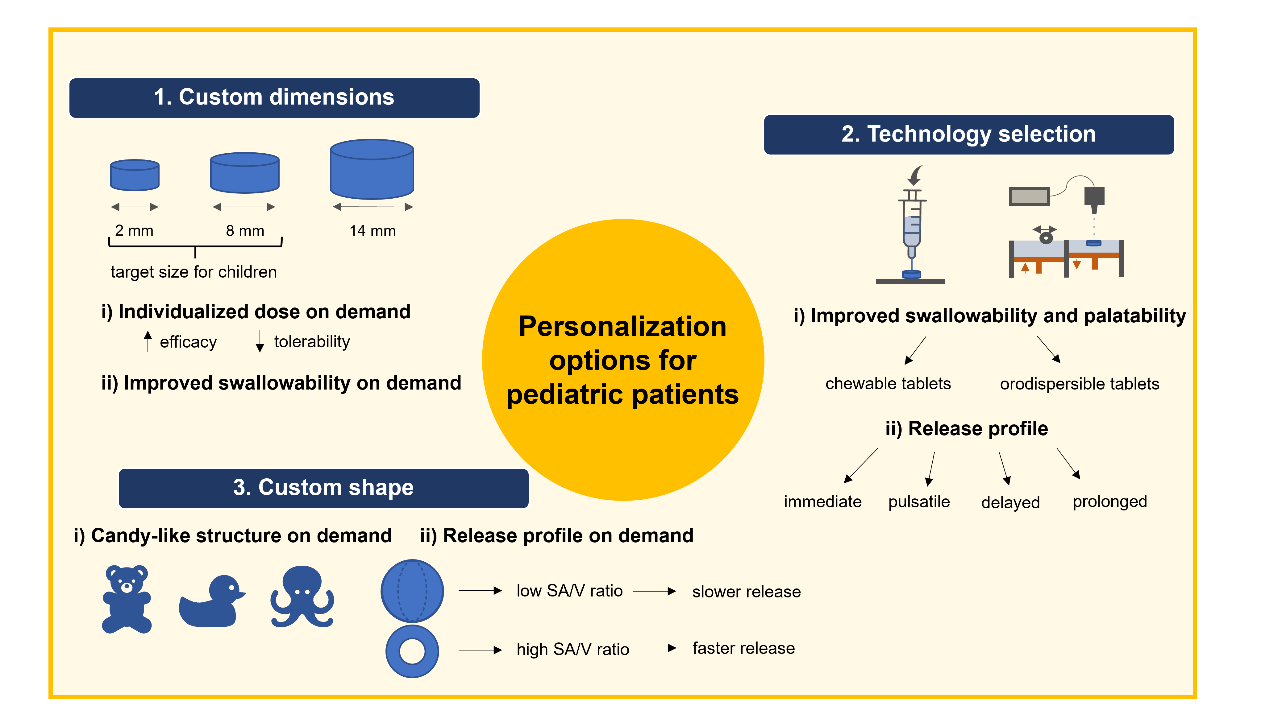
Fig. 3. Opportunities for medicine personalization for pediatric patients with 3D printing. Custom dimensions and custom shapes are available on demand at the point of care, while palatability features and release profiles should be considered beforehand in technology selection.
Shape is another on-demand customizable factor of dosage forms with a direct impact on drug release due to the change in surface area to volume ratio ( SA/V). As already extensively described in the literature, shapes with higher SA/V ratios lead to faster drug release profiles and vice versa (76, 77). By picking ring-like shapes with a higher SA/V ratio or sphere-like shapes with a lower SA/V ratio, the release profile could be tailored based on the patient’s preferences while maintaining the personalized dose (78, 79). For example, it was demonstrated that the release of paracetamol from 3D printed tablets could be customized by varying ring, mesh, and cylinder geometries (80). Moreover, dosage forms with candy-like structures could be prepared, which are familiar to children (81). In this way, adherence and swallowability could be improved due to familiarity. Fruit-shaped and animal-shaped personalized 3D printed dosage forms were already prepared with a focus on the preferences of pediatric patients (57, 82). However, caregivers should take special precautions as familiar candy-like shapes might pose a risk of misuse or overdosing for the pediatric population in case of unsupervised administrations. Access and application of medication should be strictly under the control of the caregiver, which is obligatory irrespective of the standard or 3D printed medication.
Initially, 3D printing concepts seem to be applicable for individual prescriptions of dosing schemes and by that represent small-scale batch production. However, it can also be applied for mass production as in the case of Spritam® (67, 83, 84) by forfeiting some personalization options. Consequently, 3D printing can cover both demands depending on the selected printing technology.
3D PRINTING AT POINT OF CARE
Decentralized manufacturing model
Pharmaceutical manufacturing plays an integral role in the overall drug development value chain in a centralized process. Currently, the supply chain of final dosage forms starts at the pharmaceutical company and in most cases leads to the distributor (wholesaler, pharmacy). Based on a prescription, the patient receives the medication from the pharmacist. Large-scale centralized manufacturing model is preferred to reach the global demand for established pharmaceuticals. Mass manufacturing at high production capacity ensures low cost and access to medicine worldwide. In addition, the manufacturing processes are standardized and globally available, which enables robust technology transfer between continents. While for standard dosage forms or combination products, the centralized drug-making model is superior, a more flexible and adaptable decentralized drug-making model could be used for individualized doses of 3D printed medication (10, 85–87).
In the case of a decentralized manufacturing model, the value chain of the pharmaceutical company would no longer include the production of the final dosage form (Fig. 4). Rather, the pharmaceutical company would provide the 3D printing materials together with the printing instructions and dosing recommendations to the compounding center. This information could be stored in a specific algorithm for 3D printing, which refers to a digital library of files such as the CAD file (Fig. 1), printing parameters, and even guidelines for individualized dosing calculations. The development of a specific algorithm would require an in-depth knowledge of the complex drug development process, regulatory requirements, and good practice guidelines (GxP). All highly specific printing materials including patent-protected APIs can be provided in prefilled cartridges or filaments in a secured supply chain via wholesalers. This is no different from the existing supply chain for centralized manufacturing. The novelty of the decentralized manufacturing model would be in the manufacturing process outside of the pharmaceutical company. 3D printed medication would be prepared and packaged at point-of-care by specialized pharmacies, hospital pharmacies, or even by vendors specialized in 3D printing. This would require an adaptation in quality control (QC) processes for all 3D printed products and equipment, as traditional QC approaches do not address decentralized manufacturing on a clinical site.
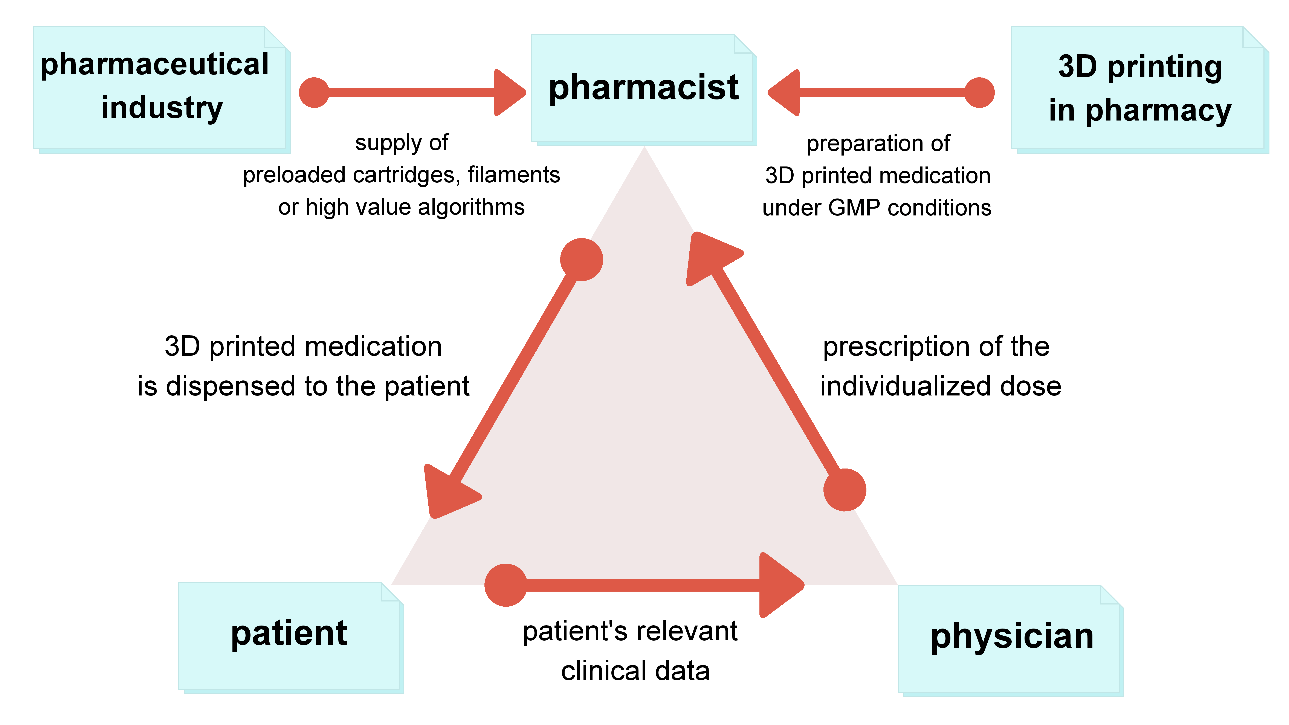
Fig. 4. Decentralized manufacturing model for 3D printed medication.
In parallel with advances in personalized medication, the relevance of patient-generated health data (PGHD) and medical data for evidence-based treatment decisions will increase (88, 89). The use of health apps and sensors for physiological parameters (fitness trackers, medical devices such as blood pressure meters, blood glucose meters, sleep detectors, etc.) and the telemetric transmission of such data to a treating physician will enable patients to provide essential input for the determination of the optimal dosing for their medication (90, 91). For example, PGHD from mobile applications were discussed to support clinical decisions to treat asthma (92). When determining optimal doses, physicians may need to consider all measured parameters. This can be achieved through the use of advanced dosing software, unless physicians are able to determine the custom doses using traditional diagnostic methods and physiological parameters, such as age, vital signs, BMI, weight, gender, metabolic state, etc.
In the proposed decentralized manufacturing model, the role of the pharmaceutical companies would change to providers of specific algorithms and supply chain management for printing materials. The role of the pharmacist might be changed to that of a facilitator, specifically to enable 3D printing under good manufacturing practice (GMP). Concepts of specialized compounding pharmacies for 3D printing purposes have already been discussed in detail (18). Furthermore, specialized vendors dedicated to 3D printing may step in to offer printing services, materials, maintenance, and other related assistance, in case pharmaceutical companies fail to cover the decentralized manufacturing model. The role of the prescriber would expand to a PGHD interpreter using state-of-the-art algorithms and other technology to determine optimal dosing for medications. Finally, the role of the patients would extend to contributors of PGHD to allow for personalized medication prescriptions.
Limitations of 3D printing at the point of care
Several limitations need to be addressed before 3D printing becomes a viable option for medication preparation at the point of care. Firstly, the creation of intellectual property (IP) can create some challenges (84). The final product would be prepared at the point of care in a compounding center, hence it would be hard for pharmaceutical companies to claim patent protection. CAD files and printing instructions can be regarded as innovative IPs. Yet once the pharmaceutical company develops a specific algorithm, it cannot be protected by patenting (93). Therefore, a new dimension is needed to protect the innovative components of the 3D printing system.
An important limitation represents the oversight and monitoring of QC for the 3D printed products (94, 95). In a decentralized manufacturing model, QC of the final dosage form is no longer under the surveillance of the pharmaceutical company but rather needs to be established by the compounding center under adequate QC standards. Consequently, the quality of final dosage forms could be assured by in-process controls accompanying the printer technology. Further process control steps need to be developed to allow the decentralized manufacturer to perform analytical testing of 3D printed medicine. Some potential solutions are discussed in the next chapter.
The ability to individualize dose selection by the physician could become a setback. The prescriber might face a challenge when selecting a personalized dose beyond the current standards such as a high and low dose prescribed in a qd (once a day), bid (twice a day), or tid (three times a day) intake regimen. Training programs for prescribers can have a crucial role in overcoming dose selection shortcomings by providing comprehensive education on the diverse possibilities and advantages associated with 3D printing (67, 96). In addition, physicians would benefit from specific dose selection software to calculate optimal dosing based on health data and physiological parameters from an individual patient. PGHD transmission from patients to the physician could represent a limitation due to data privacy. Instead, an evaluation of physiological parameters could be conducted directly in the physician's office while the patient is present. This would enable the collection of essential data for accurate dose calculations without the risk of unwanted data disclosure.
The costs associated with the 3D printed medication might represent an economic limitation, especially for the reimbursement of prescriptions by health insurance. 3D printing materials and equipment are not necessarily a high-cost driver (97). Rather, slower processing times and many individual manufacturing steps raise the total expenses. As long as highly efficacious optimal doses are used and side effects are avoided, a significant economic value for such medication can be demonstrated (98, 99). In the case of expensive APIs, a proportionally higher price of the medicine might be justified. Nevertheless, commercially viable solutions for mass production are more difficult to develop compared to the smaller scale of 3D printing.
Lastly, there are regulatory limitations that currently prevent the mass adoption of 3D printing (100). Although the FDA released the “Technical Considerations for Additive Manufactured Medical Devices: Guidance for Industry and Food and Drug Administration Staff” (101) addressing medical devices, there are no specific guidance documents yet released from any health authority about 3D printing of medicine (94,102,103). In the United Kingdom, research groups are in discussion with regulatory bodies to develop guidance documents (85). Other open questions remain from a regulatory point of view, such as the potential classification of 3D printers as medical devices, the regulatory status of the CAD file, and the regulation of a final individualized 3D printed medication (104). It might even be required to receive specific guidelines from regulators for each printing technology due to the variability between them (67). Furthermore, the current procedure of gaining health authority approval for just a few doses of a new medication might need to be adjusted. Rather, a broad dosage range would be preferred for approval of a new chemical entity for application in specialized 3D printed medication (105).
GOOD MANUFACTURING PRACTICE AND QUALITY CONTROL
Before 3D printing emerges as a new commercially viable pharmaceutical technology, QC considerations associated with the formulation and manufacturing methods should be elaborated (14, 106). With a potential shift from mass production to mass customization, innovative QC strategies are required (107). For a highly regulated pharmaceutical industry, appropriate QC methods are of critical importance due to the impact of the final product on a patient’s health and safety.
Current QC approaches are based on destructive analysis of a small sample taken from the manufactured batch. However, customized 3D printed dosage forms may potentially lead to a unique product for a single patient. In this context, current QC strategies are not feasible anymore (107). As a result, process analytical technology (PAT) and simulation approaches could be integrated into a 3D printer to advance quality assurance (QA) together with QC (108, 109). Preparation of 3D printed medication should encompass GMP, software validation, in-line process control during the printing process, non-destructive QC of the final product, cleaning validation, and QA.
Current commercial 3D printers have not been designed for GMP use in the pharmaceutical industry (86). The printer parts in contact with the formulation should be pharmaceutical grade without the potential for leaching. The cleaning process should be simple to ensure the safety of the dosage form for human use. In addition, 3D printers should guarantee traceability and data security to prevent prescription tampering or medicine counterfeiting. Software for CAD file creation and slicing needs to be validated to produce a given dosage form for selected equipment (110). A QC system should be sensitive to the vast customization features while remaining sufficiently robust across all variations within a product line.
Extemporaneous 3D printing of oral solid dosage forms requires real-time process control. PAT tools could be implemented for on-line measurements, such as mass, uniformity of mass, temperature, and pressure measurements, as well as surface scanning for the detection of defects (111). Additionally, non-destructive analysis is relevant as a QC strategy for 3D printed medication (107, 112). Simple optical imaging may provide information on the external dimensions, the surface area, overall shape, and structure of the final dosage form. Scanning electron microscopy (SEM) could be utilized to assess important quality attributes such as resolution, layer height, and surface porosities. API concentration could be quantified using near-infrared (NIR) imaging (108). To assess release profiles, novel nondestructive or nano-probing analytical methods should be developed. Currently, the high price and complexity of such technologies are limiting factors for implementation at compounding centers.
3D PRINTING FOR CLINICAL TRIALS
Dose range finding studies are usually executed during Phase IIb clinical trials. These studies are suitable for flexible dosing as a feature of 3D printed medication, which is of particular interest to the pediatric population. Dose-finding trials could be redesigned by determining a minimal and a maximum effective dose, while all doses in between would not have to be tested in a clinical trial setting. Although the regulatory acceptance of in-silico modeling still needs to be established, simulation techniques and mathematical models allow for efficacy prediction of a wide range of doses. To test some selected doses, a 3D printed tablet would be required for clinical trial supplies (84). As this represents a small-scale batch production, printing could be performed on demand and with little if any overage. In this way, 3D printing could provide more flexibility to clinical trials and accelerate pediatric medicine introduction.
There is considerable promise in adaptive dose-finding trials, in which studied doses could be changed based on accumulating data (113). One of the obstacles to realizing the full potential of these trials is the logistics of the drug supply (114). Drug manufacturing and drug supply chains are not sufficiently flexible to produce new dosage strength and resupply trial centers within feasible timeframes. Thus, the necessary drug supply for all potential adaptations is usually prepared at risk. In practice, this limits the feasibility of the number and frequency of trial adaptations. On-demand creation of dosages at trial centers – along with progress in real-time data capture from patient sensors – has the potential to optimize the adaptive dose-finding trials. The drug development stage of the product life cycle may be optimized using 3D printing. Products, that are initially 3D printed in development for dosing trials, could still be manufactured by standard processes with few dosage strengths in later stages of development before commercial launch. In case clinical data shows there is indeed a need for dose individualization, the approach of 3D printing in small batches can be kept as a commercial approach as well.
Furthermore, 3D printing would allow for faster prototyping which is relevant for first-in-human trials. Matching 3D-printed medication could be printed at the same time as placebo for clinical trials. However, academic or industry adoption cases are required as the proposed benefit otherwise remains hypothetical (17). A related review further summarizes the advantages of 3D printed oral solid dosage forms for first in human clinical trials (86). Lastly, high dose flexibility is of particular interest for pediatrics. While long-term stability can be established for any printing entry material (filaments, powder blends, etc.), only short-term stability data are required for final dosage forms. Moreover, a new variety of taste masking and blinding procedures are possible for clinical trials.
CONCLUSIONS
3D printing of oral medications might be used as a promising niche for specialized products instead of a mainstream mass-production model. The advantages of 3D printed medication have been demonstrated throughout the article. Improvements in dose personalization, shape customizations, palatability, release kinetics, and swallowability can increase adherence for pediatric patients.
Apart from the first FDA-approved 3D printed medication, a major breakthrough has not yet occurred. However, there seems to be a lot of commercial interest beyond the academic niche. Several manufacturing companies are involved in 3D printing development in the form of a product, service, or collaboration, such as Merck, Triastek, FabRx, and Aprecia (32, 83, 103, 115).
Currently, the technological limitations of 3D printing need to be surpassed as there is no single preferred manufacturing technology yet. The printing process requires completely new IP, quality, dosing, and regulatory approaches, which are not yet reflected in guidelines from health authorities especially for a decentralized manufacturing model. These bottlenecks would have to be circumvented to facilitate the adoption of 3D printing for pharmaceutical applications.
With advancements in 3D printing, the dose-finding studies in clinical trials would not be limited to dose optimization. A clinically relevant broad dose range for a given treatment could be determined. Digitization will play an important role in the adoption of 3D printing. CAD files, printing instructions, and guidelines for individualized dosing calculations will have to be interwoven to provide new opportunities for the expansion of traditional business models within the pharmaceutical value chain.
Funding. – This research received no external funding.
Conflict of interest. – The authors declare that they have no known conflict of interest. Opinions of the authors are their own, and do not represent an opinion of their employers.
Authors contributions. – Conceptualization and investigation, K.K., M.F. and V.M.; original draft preparation, K.K., M.F. and V.M; review and editing, K.K., M.F. and V.M. All the authors have read and agreed to the published version of the manuscript.