Introduction
In the dairy industry, milk is subjected to different processing conditions that affect its native equilibrium, leading to structural changes in milk proteins. Understanding these changes is essential in order to control a process and obtain a product with desirable characteristics. Milk proteins are classified in two groups including whey proteins and caseins, which represent 20 and 80% of protein in milk, respectively (O’Mahony and Fox, 2013). The main whey proteins in milk are α-lactalbumin (α-LA) and β-lactoglobulin (β-LG). These globular proteins are heat sensitive, and easily denature and aggregate when heat treatment is applied. Caseins, on the other hand, are much more heat-stable structures. Individual caseins combine into a supramolecular particles termed casein micelles. The micelle is majorly composed of colloidal calcium phosphate (CCP) and four caseins, i.e., αs 1-, αs 2- and β-casein (CN) located in the interior of the micelle and κ-CN localised on the surface of the micelle acting as a stabilizer from further aggregation (Huppertz, 2013). The individual caseins are first involved in building a primary casein particle (PCP) which is further linked with other PCPs by the calcium phosphate nanoclusters (Huppertz et al., 2017). The structure of individual caseins is adapted to their biological function (de Kruif and Holt, 2003) classifying them as naturally unstructured proteins (McMahon and Oommen, 2013). Calcium phosphate nanoclusters acting as a reinforcement are mainly bound to the phosphoserine residues of the caseins, and likely glutamate and aspartate residues, thus providing structural stability to the casein micelle (Gaucheron, 2005).
Concentration of raw milk induces subtle but important conformational changes of the milk proteins (Markoska et al., 2019a), which are further augmented by heating (Markoska et al., 2019b). These changes include dissociation of individual caseins from the micelle, the formation of aggregates of serum and micellar caseins with whey proteins. Concentration of milk also decreases pH by 0.2 or 0.3 units for a concentration factor of 1.9 or 2.8, respectively (Markoska et al., 2019a). Heat treatment of concentrated milk further reduces pH due to generation of organic acids from lactose degradation and shifting of calcium and phosphate from the serum into the micelle forming insoluble calcium phosphate, which is accompanied with release of hydrogen ions; these pH reductions can destabilize the casein micelle and lead to protein aggregation (Singh, 2004). While κ-CN dissociation is proportionally affected by concentration factor and temperature, other caseins appear to frequently relocate between two phases which may be related to a shift in a mineral balance under these conditions (Markoska et al., 2019b).
After concentration of milk, the particles in the milk are found in a new equilibrium with (more) closely packed molecules with reduced electrostatic repulsion due to elevated ionic strength, thus facilitating interactions upon heating. In addition, stability of concentrated milk systems to heat-induced coagulation is lower compared to unconcentrated milk, and is maximal in the pH range between 6.4-6.8 (Huppertz, 2016; O’Connell and Fox, 2003; Singh, 2004). Any alteration of milk pH prior to heat treatment can impact its heat stability. Increasing pH increases the negative charge of the caseins, which can lead to a loose and expanded micellar structure, leading to swelling of the micelle at a pH of up to 8.5, above which the micelle appears to disintegrate (Sinaga et al., 2017).
While the effect of milk concentration and pH on macroscopic effects of heat treatment, in terms of milk coagulation and protein aggregation, have been widely studied, less is known how these observations are related to conformational changes in milk proteins. The current work follows on our previous observations on structural and compositional changes in milk systems upon concentration observed using an ATR-FTIR and SDS-PAGE electrophoresis (Markoska et al., 2019a, b), now including pH adjustment as an additional factor. FTIR was shown to be applicable method for structural studies of milk proteins due to its sensitivity to changes in peptide interactions being covalent or non-covalent bonds (hydrophobic and electrostatic) (Grewal et al 2017b). The current study aimed at elaborating further on observed instabilities of concentrated milk systems upon pH readjustment and heating to and holding at 121 °C.
Materials and methods
Sample preparation
Freshly collected raw milk from Murray Goulburn Cooperative Co. Ltd (Laverton North, Victoria, Australia) was supplemented for experimental purposes by adding sodium azide (0.01 %) for prevention of bacterial growth. The milk was skimmed by centrifugation at 40 °C for 30 min at 3225 g (Avanti J-26XP, Beckman instrument Australia Pty. Ltd, Gladesville, NSW, Australia). Preparation of the milk samples with different solids level (9, 17 or 25 % total solids-TS) was conducted according to Markoska et al. (2019a). In addition, the concentration was performed by evaporation at 55 °C up to the required levels using a rotary evaporator (Rotavapor® R100, John Morris Scientific, Deepdene, Victoria, Australia). The pH of each sample was measured using a pH meter (Metrohm AG, Herisau, Switzerland) and subsamples were adjusted to pH of 7.5 by dropwise addition of 6M NaOH with a continuous stirring. After pH adjustment, the samples were placed in an oil bath set at 121°C and then removed one by one when temperature reached 75, 85, 95, 100 or 110 ºC (representing 0 s holding time), while the final sample was kept at 121 ºC for 2.6 min (to satisfy a 12D concept) as described by Markoska et al. (2019b). The final heating stage resembles the minimum conditions used for sterilization of evaporated milk in industrial practice required to deactivate the spores of Clostridium botulinum (Early, 1998). The average heating rate was calculated to be 1.12 °C/s. After treatment every sample was immediately cooled in an ice bath to room temperature. The treatment and the analysis were performed in duplicate from two different batches of milk collected on separate occasions.
Determination of non-sedimentable protein
Separation of the serum phase was performed by centrifugation at 21 °C for 1 h at 100,000xg using a Beckman Ultra L-70 centrifuge (Beckman Coulter, Australia Pty. Ltd, Gladesville, NSW, Australia). Changes in the protein composition in the serum phase were assessed using SDS-PAGE under reducing and non-reducing conditions as described by Grewal et al. (2017a). Each sample was diluted in a SDS-PAGE buffer containing 0.0625M Tris-HCl buffer, 10 % [vol/vol] glycerol, 2.5 % [vol/vol] of 0.4 % [wt/vol] bromophenol blue solution and 20 % [vol/vol] of 10 % [wt/vol] SDS. From the prepared samples, exactly 12 μL was loaded into 12.5 % gels and run using a buffer containing 0.1 % SDS, 0.025 M Tris, and 0.191 M glycine at pH 8.6. The electrophoretic parameters were 85 min at 210V and 70mA in a Protean II xi cell (Bio-Rad Laboratories). The image for each gel was conducted using ChemiDoc imager (Chemidoc MP; Bio-Rad Laboratories). For the reducing gels, exactly 20 μL of β- mercaptoethanol was used as a reducing agent to separate formed aggregates as a result of applied treatment. For evaluating the impact of pH adjustment on behaviour of proteins, only non-reduced SDS-PAGE gels were used to avoid data overcrowding since the changes were minimal. For each concentration, one gel set is shown presenting the impact of treatments at both pH levels. The temperature treatment is, on the other hand, presented under both reducing and non-reducing conditions for more transparent band interpretation. In addition, one set for each concentration level observing the temperature effect on samples with altered pH to 7.5 was prepared. The band intensity of the SDS PAGE on heat treated samples was analysed using Image Lab software (Bio-Rad Laboratories). Each band was quantified to observe the difference in the protein content as a result of the applied treatments.
Fourier transform infrared (FTIR) spectroscopy
Immediately after cooling, the samples were analysed for changes in protein conformation using a PerkinElmer FTIR spectrometer (Frontier, PerkinElmer, Boston, MA, USA). Measurements were carried out in the range of 4000-600 cm -1 with a resolution of 4 cm -1 and averaging 16 scans for each spectrum as described by Grewal et al., (2017b). Water was used as a background spectrum scanned at the beginning of the measurements using blank diamond ATR cell. The absorption of the bands of water in region 1730-1600 cm -1 (H-O-H bending vibrations) were overcome by water subtraction and baseline correction (Grewal et al., 2017b). Spectragryph (software version 1.2.11, Oberstdorf, Germany) was used for calculation of second derivative or a negative band in the region 1700-1600 cm -1 (Amide I) for greater resolution of the peaks.
Results
Changes in SDS-PAGE patterns
The partitioning of proteins between the colloidal and serum phases resulting from the pH adjustment was observed in the SDS-PAGE patterns of the serum phase of the samples under non-reducing conditions (Figure 1). The effect of pH adjustment is shown in Figure 1. In unconcentrated milk (9% TS) when pH was increased to 7.5 a slight increase in band intensities was observed for the α s-CNs and β-CN in the serum. A similar, but less intensive, trend was observed in the samples with 17 % TS upon pH adjustment. However, at 25 %TS no major changes in the intensities of the protein bands were observed (Figure 1).
Heating of the samples at pH 7.5 resulted in great concentration variations of whey proteins and caseins in the serum. As presented in the SDS-PAGE patterns in Figure 2, the applied heat reduced the concentration of whey proteins in the serum with increasing temperature, with major reductions taking place at 110 and 121 ºC. The intensity of reduction varied depending on the concentration levels and obviously heating intensity. In unconcentrated milk adjusted to pH 7.5, heat treatment caused increase of serum κ-, α s- and β-CN concentrations immediately upon temperature reaching 75 °C, while the β-LG and α-LA bands were less intense at the same time. The patterns under reducing conditions confirmed creation of serum aggregates between κ-CN and β-LG at all heating levels (Figure 2A,D). The created aggregates varied in their molecular size as observed in their distribution in the SDS-PAGE gels. Moreover, these aggregates disappeared after the bonds were reduced and large whey proteins including immunoglobulins and BSA appeared. Considering their low concentration in milk, their role was not assessed in the current work but should not be discounted. Upon prolonged heating at 121°C, the concentration of α-LA in the serum decreased and it appeared involved in aggregation reactions with β-LG resulting in serum and micellar aggregates. The presence of aggregates was confirmed at the top of the lanes in the non-reducing SDS-PAGE patterns (Figure 2A). The observed aggregates after heating at 121 °C increased by 78 % in comparison to the non-heat treated sample.
In milk with 17 % TS during heating up to 110 °C, the levels of κ-CN increased in the serum consequently leading to complexation with β-LG (Figure 2E). The level of α s- and β- CN did not change substantially in the serum. The concentration of monomeric α-LA and β-LG in the serum started to decrease intensively from 85-95 °C. In addition, these whey proteins were observed to form aggregates with caseins (mainly κ-casein), which become more prominently present in the serum as temperature rose reaching 43% increase at the final heating stage. As presented in the non-reducing SDS-PAGE gels (Figure 2B), the band associated with α-LA almost disappeared due to aggregation. After heat treatment at 121°C, α-LA, in addition to formation of serum aggregates with β-LG and κ-CN, also appeared to be involved in formation of insoluble large complexes that sedimented on ultracentrifugation. The observed aggregate intensities were 91 % greater than those in the control samples.
When milk concentrated to 25 % TS was heated up to 110 °C, the concentration of whey proteins and caseins in the serum (Figure 2C,F) followed a similar but more intense trend as in milk concentrated to 17 % TS (Figure 2B,E). Thus, the micellar dissociation of caseins and aggregation of denatured whey proteins started at lower temperature point and continued intensively as temperature rose. This was followed by formation of serum aggregates of denatured whey proteins and dissociated κ-CN, as evidenced on the top of the stacking gel (Figure 2C). After final heating, the band intensity associated with the serum aggregates was 93 % greater than that in the non-heat treated sample. However, after prolonged heating at 121 °C, a part of the formed serum aggregates appeared strongly bonded since the reducing reagent could not separate them and these aggregates remained at the top of the stacking gel (Figure 2F). Another interesting point after prolonged heating at 121 °C was the involvement of serum α s-CN and β-CN in aggregation along with the κ-CN and whey proteins.
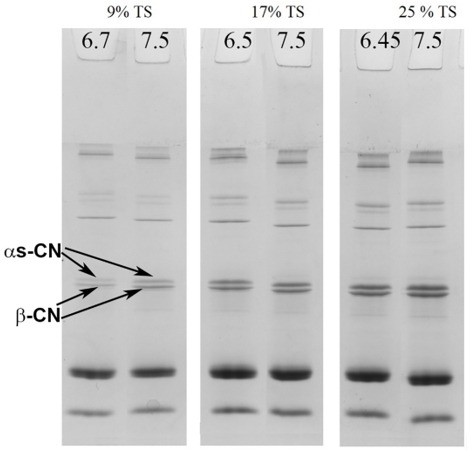
Figure 1. SDS-PAGE under non-reducing conditions of serum phase of unheated skim milk samples containing samples with 9, 17 or 25% total solid at their natural pH and with pH adjusted to 7.5
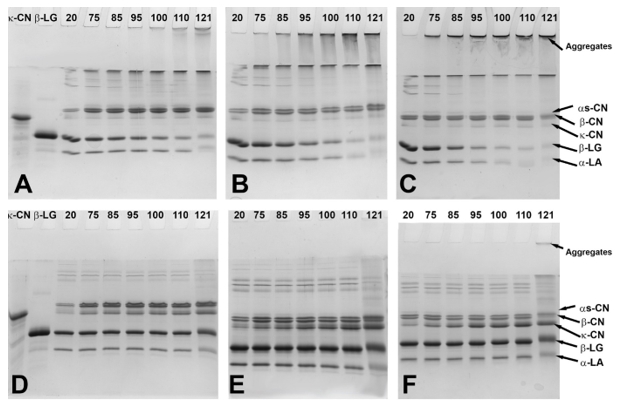
Figure 2. SDS-PAGE under non-reducing (A-C) and reducing (D-F) conditions containing control and heated samples with 9 (A,D), 17 (B,E) or 25 (C,F)% total solids. The values from 20 to 121 represent the temperature at which the samples were removed from the oil bath
Changes in FTIR patterns
The secondary structure of proteins was observed by FTIR in the region of 1700-1600 cm -1 (Amide I) related to C=O stretching vibrations of the peptide bonds (Kher et al., 2007). The effect of temperature on the secondary structure of proteins in the samples with adjusted pH to 7.5 was observed for the samples obtained after heating at 110 and 121ºC compared to the control sample (Figure 3). This temperature range was selected based on the fact that most of the structural changes were observed at these temperatures and for the sake of simplification of the overcrowded spectra.
When the pH value of unconcentrated milk was adjusted to 7.5, the major observed change in the second derivative of the spectra was a decline in random structures (1640 cm -1) (Figure 3A). Moreover, temperature treatment up to 110 °C resulted in appearance of new peaks at 1618 cm ‑1 (intermolecular β-sheets) and 1698 cm -1 (β-sheet aggregates) and increase in a peak at 1653 cm -1 (α-helix-loops). After prolonged heating at 121 °C, the peak at 1653 cm -1 returned to almost original state prior to heating, similarly the newly formed peaks at 1618 cm -1 rearranged into nearly same position as in unheated milk.
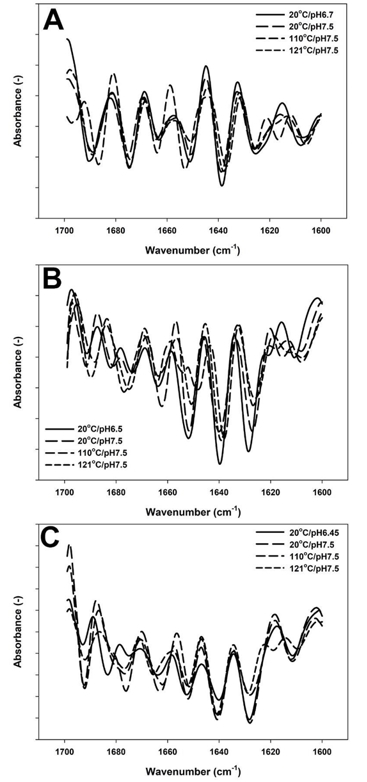
Figure 3. Second derivative of Amide I region spectrum of skim milk samples with 9 (A), 17 (B) and 25 %TS (C). Control with native pH ( ), pH adjusted to 7.5 ( ), treated at pH 7.5 at 110°C/0min ( ) and treated at pH7.5 at 121°C/2.6min ( )
In the samples with 17 % TS, in addition to a reduction of a peak at 1640 cm -1, as previously observed for 9 % TS, an increase in turn structures (1663 cm -1) and appearance of a new peak at 1618 cm -1 was noted when milk was adjusted to 7.5 (Figure 3B). Next, heating up to 110 °C exhibited a decrease in intramolecular β-sheets (1628 cm -1), reformation of intermolecular β-sheets (1618 cm -1), increase in a peak at 1678 cm -1 and two new peaks were formed at 1649 and 1655 cm -1 associated with new α-helical structures (Figure 3B). However, when the system was further heated to 121°C, FTIR spectra exhibited a loss of a peak at 1618 cm -1 (intermolecular β-sheets), greater peak intensity at 1690 cm -1 (aggregated β-sheets) and the peak at 1652 cm -1 reappeared but with a lower intensity in comparison to that of the unheated samples.
In the sample with 25 % TS, the pH alteration induced an increase in intensity of a peak at 1640 cm -1 (random structures) followed by intense reformation of new β-turn structures (1663 and 1675 cm -1) (Figure 3C). Heat treatment up to 110°C resulted in intense spectral variations including a decrease in a peak intensity related to intramolecular β-sheets (1628 cm -1), followed by extending of a peak loop from 1678 to 1680 cm -1 (Figure 3C). After heating at 121 °C for 2.6 min, reappearance of a low intensity peak at 1618 cm -1 (intermolecular β-sheets), decrease of antiparallel β-sheets (1628 cm -1) and shifting of a peak at 1663 to 1667 cm -1 (β-turn) were predominant changes.
Discussion
In our previous work we observed protein partioning between the serum and colloidal phases using the same concentrations and temperature range as in this study but at native (not adjusted) pH (Markoska et al., 2019b). It was shown that the most notable changes were in intermolecular β-sheets of β-LG, reformation of α-helix of α-LA at 121 °C and increased random structures due to involvement of κ-CN in aggregates with whey proteins. In the current work the changes in the secondary structure of proteins occurred as a result of pH adjustment of the samples which continued during heating.
The pH effect on the protein structure was more pronounced as the concentration factor increased, indicating intensive protein restructuring before heating. In addition, the pH-adjusted system before heating already created new hydrogen bonds among the caseins in the micelle or serum (Deeth and Lewis, 2016; Farrell et al., 2001). These resulted from the extension of the polypeptide chains and rearrangement of the random and turn structures of the caseins or swelling of the casein micelle at elevated, alkaline pH (Vaia et al., 2006). Thus, the observed restructuring in the random structure in the FTIR spectra at all concentrations confirms rearrangement of the natural folding of the proteins in the system. The casein micelles remain stable, since no serum increase of κ- CN was noted as pH increased, which is known to be external micellar stabilizer (Huppertz 2013). Hence, the observed slight dissociation of β- and α s-CN from the micelle in unconcentrated milk sample can be related to the disruption of hydrogen and electrostatic bonds that keep these caseins connected to the neighbouring caseins. However, this dissociation was not observed in more concentrated systems, most probably as particles have less mobility in the crowded environment.
Temperature had a more pronounced effect on the pH-adjusted samples containing elevated solid concentrations. For the assessed concentrations, during heating up to 110 °C, the most affected structures were intra- and intermolecular β-sheets. These changes were previously confirmed to correspond to aggregation of denatured β-LG (Lefèvre, and Subirade, 1999; Markoska et al., 2019b). Created serum complexes in the unconcentrated milk at this temperature were only covalently bound structures of β-LG and dissociated caseins (predominately κ-CN). However, as the concentration factor and temperature increased, α-LA engaged in aggregate formation. This protein is fairly stable in the unconcentrated environment. Anema (2001) observed that denaturation of α-LA is hardly affected by concentration. However, the heat induced denaturation of this protein can be high under specific conditions such as high pH observed in our study. Moreover, when heating is applied at elevated pH, increased net negative charge of the molecules leads to greater intermolecular electrostatic repulsions, which stimulate unfolding and aggregations of the proteins (Law and Leaver, 2000). At higher solid concentration, the particles are closely packed so the reactions can take place at lower heating temperatures than in unconcentrated environment. Since α-LA has no tendency for self-association it can only aggregate through disulphide interchange reactions with the free thiol group of β-LG (Livney et al., 2003; Mediwaththe et al., 2018) which also followed similar trend depicting the aggregation dependence of pH and temperature. At all observed concentrations heated up to 110°C, the formed aggregates were serum structures composed predominately of β-LG-α-LA-κ-CN moieties. In addition, these complexes are created when elevated temperatures are applied promoting disulphide bonding between κ-CN and β-LG and disulphide interchange reactions between α-LA and β-LG (Markoska et al, 2019b; Anema, 2001)
Prolonged heating at 121 °C continued the previous aggregation trend. At this step, the aggregation was more intense which was expected due to intense temperature treatment and prolonged holding time. At this step, the heating was extended to 2.6 min which is the reason for more intense aggregation. During heat treatment the aggregate formation increased as the concentration factor increased from 9 to 17 and to 25 % TS. Moreover, at all observed concentrations, β-LG and α-LA were almost completely involved in aggregation, which was confirmed by reformation of β-sheets and α-helical structures, respectively. At this stage even after cleaving most of the covalent bonds created via disulphide bridging, a part of the serum aggregates in milk with 25 % TS did not separate into individual proteins, which was likely due to lactosylation via Maillard reaction due to intense heating. These aggregates, while large, were still very soluble and did not precipitate upon centrifugation. During this step, in addition to serum aggregates, large complexes with the casein micelle were formed which precipitated in the pellet after ultracentrifugation. These complexes in the samples containing 25 % TS, in addition to previously described structure consisting of β-LG-α-LA-κ-CN, also involved dissociated serum α s- and β- casein, which interacted via hydrophobic interactions due to presence of distinct polar and hydrophobic domains (McMahon and Oommen, 2013). This was also confirmed by formation of new β-turn structures, which may be related to greater involvement of caseins in the formed aggregates.
Conclusion
The current study depicted the changes in the structure and positioning of the proteins between colloidal and serum phases as some of the main reasons for low heat coagulation time (HCT) of concentrated milk upon pH adjustment. Proteins undergo structural reformation as a result of alkalization that facilitates further aggregation during heating. The main reason for this instability appears to be intensive aggregation of whey proteins with the caseins, which leads to formation of large aggregates that become strongly bonded as the concentration factor increased. In our previous study (Markoska et al., 2019b) we concluded that pre-warming of milk would denature whey proteins and prevent further aggregation with caseins, thus stabilise the system. However, the current study shows that this approach may not be sufficient to prevent this process since the caseins promote more intense structural modifications as a result of pH and applied heat treatment and overall their involvement in formation of various complexes. As it appears, elevating pH disrupts the native equilibrium of the caseins leading to rearrangement of their secondary structure and formation of new hydrogen and strong covalent bonds in highly concentrated environment leading to low heat stability at this pH.
Utjecaj pH vrijednosti i udjela suhe tvari na strukturu proteina kao funkciju toplinske obrade mlijeka
Sažetak
Sustavi koncentriranog mlijeka vrlo su nestabilni tijekom toplinske obrade pri svom prirodnom pH. U ovom su radu identificirane strukturne reakcije proteina zajedno s izmjenama sastava koje su se dogodile tijekom grijanja i uzorkovanja na 75, 85, 95 100, 110 i 121 °C sirovog obranog mlijeka koje sadrži različite udjele ukupne suhe tvari i pH prilagođenog na vrijednost 7,5. Sirovo mlijeko je koncentrirano na 17 ili 25 % ukupne suhe tvari, nakon čega je pH podešan na 7,5. Promjene u strukturi proteina utvrđene su pomoću Fourierove transformirane infracrvene spektroskopije (FTIR), a razdioba proteina između serumske i micelarne faze proučena je pomoću SDS-PAGE elektroforeze. Podešavanje pH vrijednosti rezultiralo je laganom disocijacijom kazeina u serumu, pretežno u kontrolnim nekoncentriranim uzorcima. Podešavanje pH vrijednosti je odmatanja proteina rezultiralo povećanjem slučajnih struktura u svim uzorcima koncentriranog mlijeka. Toplinska obrada značajno je utjecala na sastav proteina u serumskoj fazi te izazvala strukturne modifikacije. Svi uzorci mlijeka zagrijani do 110 ° C pokazali su gubitak topljivih frakcija β-LG, α-LA i kazeina (uglavnom κ-kazeina) što je najvjerojatnije rezultat agregacije uzrokovane toplinom. Intenzitet agregacije se povećavao s faktorom koncentriranja. Sličan trend je utvrđen i na 121 °C, ali s većim agregatima. Kako se faktor koncentriranja povećavao, počele su prevladavati kovalentne veze među novoformiranim strukturama.
Ključne riječi: koncentrirano mlijeko; toplinska obrada; struktura proteina; podjela proteina
References
https://doi.org/10.1111/j.1365-2621.2001.tb15573.x .
Deeth, H., Lewis, M. (2016). Protein stability in sterilised milk and milk products. In Advanced dairy chemistry (pp. 247-286). Springer, New York, NY.
https://doi.org/10.1007/978-1-4939-2800-2_10
De Kruif, C.G., Holt, C. (2003): Casein micelle structure, functions and interactions. In Advanced dairy chemistry-1 proteins (pp. 233-276). Springer, Boston, MA.
https://doi.org/10.1007/978-1-4419-8602-3_5
https://doi.org/10.1016/s0268-005x(01)00080-7
https://doi.org/10.3168/jds.2016-11278
https://doi.org/10.1016/j.idairyj.2016.11.014
https://doi.org/10.1007/978-1-4614-4714-6_4
https://doi.org/10.1007/978-1-4939-2800-2_7
https://doi.org/10.1016/j.idairyj.2017.03.006
https://doi.org/10.1016/j.vibspec.2007.03.006
https://doi.org/10.1021/jf981302b
https://doi.org/10.1046/j.1365-2621.1999.00311.x
https://doi.org/10.1021/jf034582q
https://doi.org/10.1016/j.lwt.2018.12.011
https://doi.org/10.1016/j.idairyj.2018.08.010
https://doi.org/10.1007/978-1-4614-4714-6_2
https://doi.org/10.1007/978-1-4614-4714-6_6
https://doi.org/10.1016/j.idairyj.2017.09.002
https://doi.org/10.1007/978-1-4419-8602-3_25
https://doi.org/10.1080/10942912.2016.1152480