INTRODUCTION
Monoclonal antibodies (mAb) are extensively utilized in the treatment of various diseases, including inflammatory conditions and autoimmune disorders, as well as cancer (1). Therefore, ensuring the long-term stability of mAbs is crucial in the development of biologic drugs development.
They are susceptible to both chemical and physical degradation processes. Chemical degradation examples are hydrolytic reactions (e.g., deamidation, Asp isomerization, Trp hydrolysis, and proteolysis), oxidation (e.g., metal-catalyzed oxidation, photooxidation), and condensation. On the other hand, physical stability can be categorized into two aspects: structural stability, which refers to the protein's resistance to unfolding forces (in thermodynamics free energy of unfolding) (2), and colloidal stability, which pertains to the protein's ability to remain in monomeric native form. Various stressors, such as heat, surface exposure, shear forces, light, dehydration, freezing, cold denaturation, pH, and pressure, can lead to the physical degradation of proteins (3).
Protein instability is a common concern in biopharmaceutical formulations, primarily manifested as the formation of protein aggregates, which can occur in both reversible and irreversible forms. The latter can give rise to smaller soluble particles, predominantly of interest during the early stages of development, as well as larger insoluble particles known as protein precipitates. These precipitates can range in size from subvisible to visible (4). Analyzing them is a challenge due to their low concentrations, often requiring large sample volumes for analysis. The formation of aggregates not only diminishes antibody activity but also has adverse effects on patients by eliciting unwanted immunogenic responses (5, 6). Additionally, the presence of aggregates can impact regulatory approval processes and affect delivery methods.
Aggregation of mAbs is a complex process, addressed by Basle et al. and Chi et al. (7, 8). The balance between conformational stability, , and colloidal stability, , must be achieved, to prevent aggregation. Not only characteristics of protein, but also the environment, such as excipients, pH, handling, and contaminants, have a significant impact and are usually in interplay during aggregation processes.
In the context of our study focusing on the early phase of biopharmaceutical formulation development, we specifically examined small soluble aggregates. These are typically detected using size exclusion chromatography (SEC), a technique that separates protein species based on their hydrodynamic size under native conditions, enabling the detection of soluble aggregates (multimers) and clippings (3). Other size variants, such as sub-visible and visible particles, can be detected using techniques such as micro-flow imaging (MFI) or light obscuration (LO).
Applying the above-mentioned knowledge, the optimal strategy to stabilize the formulation involves the elimination of chemical instabilities, enhancing conformational stability, improvement of colloidal stability, and optimization of interfacial stability, which can be achieved effectively using excipients (9).
Buffers, or buffer systems, play a crucial role as one of the most important components in formulations. Their primary responsibility is to maintain the pH stability of pharmaceutical products, which is vital for preserving the chemical integrity and higher-order structure (HOS) of mAb. Additionally, buffers can influence conformational stability, alter colloidal stability, and impact the proteins' behavior at interfaces.
A buffer system is a solution that resists a change in pH when acids or bases are added. It consists of a weak or moderately strong acid (or base) and its corresponding salt in a similar concentration (10). Buffer systems possess two crucial properties: buffer capacity and ionic strength. Buffer capacity is defined as the concentration of acid or base that must be added to induce a pH change and can expressed by Equation 1:
(1)
where represents the concentration of the added base, and the concentration of the added acid (11). On the other hand, ionic strength is defined as
(2)
where is the molar concentration and is the valence of ionic species . It has been shown that the ionic solution containing the protein affects its stability (12). However, it's important to note that pH stabilization alone should not be the sole consideration when selecting buffers and additional factors should be taken into account.
The effects of buffers on protein stability have been studied in detail by Ugwu and Shireesh (13) and Zbacnik et al. (14). Through a comprehensive assessment of various stabilization mechanisms, they concluded that ligand binding, which involves direct interaction between the excipient and the protein, affects both conformational and colloidal stability, while solute exclusion exclusively impacts conformational stability.
Acidic and basic excipients, providing the main buffering capacity to the buffer system, can be used as main buffer components (MBCs), with histidine, phosphate, and acetate being among the most popular choices. Table S1 in supplementary materials provides information on several buffers, along with their pKa values and chemical structures.
Zbacnik et al. (14) have already summarized several case studies investigating the effects of histidine, phosphate, Tris, acetate, and other buffer components on proteins in formulations. The objective of this study was to comprehensively characterize the suitability of various alternative buffer systems in formulations of therapeutic monoclonal antibodies against commonly used ones. Specifically, the investigation aimed to assess the performance of these buffer systems under different conditions, including varying buffer concentrations, ionic strengths, buffer counterions, mAb concentrations, and stress conditions. To ensure a focused evaluation of only the buffer's impact on mAb stability, the formulations were deliberately designed to consist solely of protein and buffer components.
EXPERIMENTAL
Excipients
The following excipients were obtained from Sigma-Aldrich (now Merck-KgaA): Acetic acid (glacial, 100 %), bis-TRIS, citric acid, D-glucuronic acid, DL-malic acid, DL-tartaric acid, hydrochloric acid (25 %, m/m), L-arginine, L-glutamic acid, L-histidine, L-lysine, maleic acid, meglumine, methanesulfonic acid, ortho-phosphoric acid (85 %, m/m), sodium hydroxide pellets, TRIS base.
Proteins
Seven therapeutic proteins, referred to as mAb-1 to mAb-7 in this study, were provided by Lek d.d. (Mengeš, Slovenia). The disclosure of mAb-1, Infliximab, and mAb-7, Rituximab, was approved, while the remaining proteins could not be disclosed due to their ongoing development process at Novartis. All mAbs are of IgG1 class, with the exception of mAb-5, which is IgG2.
Sample preparation
The mAb solutions provided consisted of mAb and buffer. For this reason, the protein material was first dialyzed in ultrapure water. The pH of the protein stocks in water was adjusted to a target pH using low concentration HCl or NaOH (with no adverse effects on the proteins as confirmed by size exclusion chromatography). Stocks were then concentrated to 150 mg mL–1 in Amicon tubes (Amicon Ultra centrifuge filter unit, 10, 30, or 50 kDa MWCO). Concentration was measured in triplicate after dilution to approximately 1 mg mL–1. Highly concentrated stock solutions of buffers (200 mmol L–1) were then prepared. Ultrapure water was used to prepare the buffer stocks. The required amounts of buffer compounds for each buffer system were calculated using ChemBuddy BufferMaker software. The pH of the buffer stock solutions was adjusted with a high concentration of HCl/NaOH, if necessary. Both the protein and buffer stock solutions were concentrated to the higher concentration required so that the final sample had the target protein and buffer concentration after compounding.
The final formulations were prepared by thoroughly mixing the components within 96 deep-well plates using the Tecan Robot Liquid Handler. Samples were then transferred to a sterilized »sandwich system« consisting of a 96 deep-well plate (collection plate) at the bottom and AcroPrep™ Advance 96-well filter plates at the top. The sandwich was then centrifuged and transferred to the UDF chamber. Filtered samples in the bottom plate were transferred to Matrix™ 2D barcoded glass storage tubes for temperature stress or Nunc™ cryogenic tubes for freeze/thaw stress. Workflow sample preparation is depicted in Fig. 1.
Stress conditions
The samples were subjected to various stress conditions. Temperature stress involved incubation in Kambič Performance Oven SP-105C for 4 weeks at 25 °C, 12 weeks at 25 °C or 4 weeks at 40 °C. Light stress was performed in the Atlas MTT Suntest XLS+ light stress chamber for 22 hours on 55 klux using Xenon Arc Lamp and ID65 solar filter. Freeze/thaw stress was induced by subjecting the samples to 10 cycles of transfer between a freezer (–80 °C) and a cold room (2–8 °C.)
Aggregate content measurement
Samples were analyzed at 40 °C on a Waters ACQUITY UPLC system using an SEC column (200 Å pore size, 1.7 μm bead size, and 4.6 mm × 150 mm column dimensions). The sample load was 0.75 μL. The flow rate of the mobile phase (50 mmol L–1 sodium dihydrogen phosphate and 400 mmol L–1 sodium perchlorate, pH 6.0) was 0.4 mL min–1 for a total run time of 5 minutes. Samples were diluted to 1 mg mL–1 in 150 mmol L–1 sodium phosphate, pH 7, and maintained at 2–8 °C in the autosampler prior to injection. Data were analyzed using Empower 3 software. The variability of the relative aggregate content measurement (aggregates/monomer) at the described column loading was estimated to be 0.05 %. Samples were measured within a linear range of detection by application of sample for analysis (0.75 µL with 1 mg mL–1 protein concentration), which follows the standard established analytical method in Novartis. Only peaks above LOQ were integrated. By following the same procedure upper limit was not reached. The percentage of aggregates in each sample was determined by integrating the peak area of the high molecular weight species (HMW) and dividing it by the sum of the peak areas for all peaks, including the main protein peak and low molecular weight species (LMW). The growth of aggregates was quantified by subtracting the percentage of aggregates measured after specific stress conditions from the percentage measured at the initial time point (). The variability of both measurements was considered when calculating the final percentage of aggregates, resulting in an overall error of 0.0707 %. To improve the clarity of the figures, error bars are not shown on the charts.
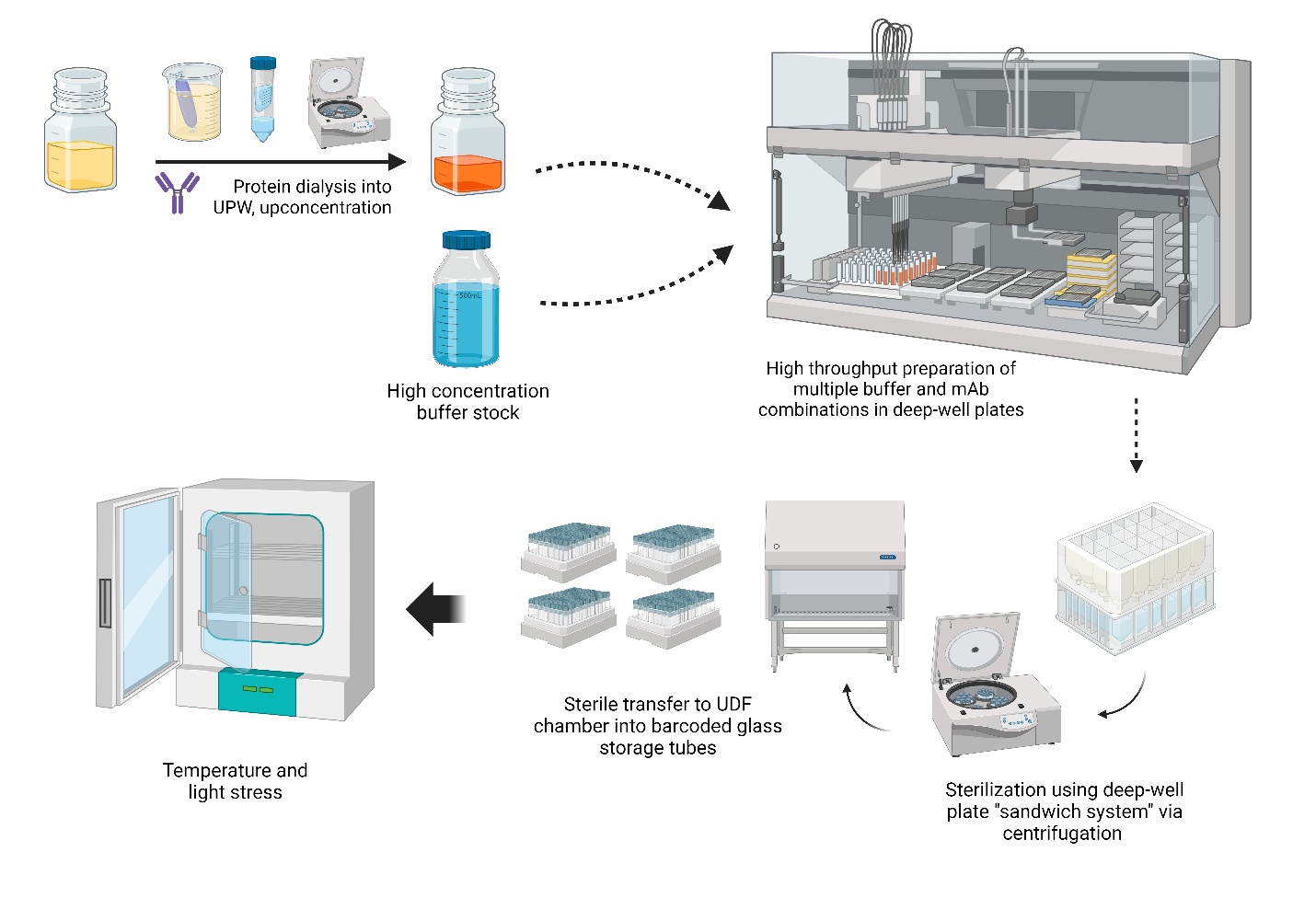
Fig. 1. High-throughput sample preparation. Upconcentration of mAb solution and preparation of highly concentrated buffer stocks combined with Tecan Robot Liquid Handler allowed for rapid compounding of numerous samples. 96 well-plate filter “sandwich” further enabled the preparation of sterile samples.
RESULTS AND DISCUSSION
Buffer systems with the same ionic strength at mAb concentration of 1 mg mL–1
In the initial phase of our study, we investigated the behavior of seven monoclonal antibodies (referred to as mAb-1 to 7) in the presence of 14 different buffers. These buffers, with a consistent ionic strength of 0.028 mmol L–1 and a pH of 6, are listed in Table I. Separate samples of each formulation were subjected to 10 cycles of freeze-thaw stress, four weeks at 25 °C, 12 weeks at 25 °C, and four weeks at 40 °C. The difference in aggregate content before (t0) and after each stress period was determined. Reference buffers were employed for comparison purposes alongside each mAb.
Table I. Buffers employed for stability testing of seven mAbs at a mAb concentration of 1 mg mL–1. The MBCs are highlighted in bold in the first column
Freeze-thaw
Following 10 freeze-thaw cycles, we observed different tendencies of tested mAbs for soluble aggregate formation in the tested buffers (Fig. 2 and Table S2). mAbs-3 and 6 exhibited the highest levels of aggregation, while mAbs-2, 4, and 7 were also affected, albeit to a lesser extent. Conversely, mAbs-1 and 5 showed minimal aggregation. Notably, histidine/HCl, despite its widespread use in biopharmaceutical formulations, proved to be one of the least favorable buffer systems for all tested mAbs. Counterions were found to play a crucial role in aggregate formation during freeze-thaw stress. Substituting chloride ions with aspartate, glutamate, or acetate as counterions in combination with histidine significantly reduced aggregate formation, sometimes by more than half. Glucuronate, as a counterion, only displayed slightly better performance than chloride. Even the buffers used in the reference drug product did not consistently exhibit the best properties in terms of aggregation propensity during freeze-thaw stress. Among all buffer systems tested, the reference buffers for mAbs-2, 3, and 5 resulted in the highest aggregate formations with their corresponding protein. No specific trend was observed for other buffers. Bis-TRIS buffers showed excellent protection against aggregate formation for some mAbs (namely 2, 4, 5, 6, and 7), while they proved highly unstable for others (1 and 3). Additionally, the use of glucuronate as a counterion with bis-TRIS buffer was not always optimal compared to other counterions tested (mAb 3, 5, 6, and 7). Sodium acetate buffer, as a popular conventionally used buffer, also proved to be a suboptimal buffer of choice for multiple mAbs. Among all the tested buffers, arginine/citrate demonstrated the best overall performance in terms of freeze-thaw stress resilience.
To interpret different stabilization of the tested buffers, the molecular mechanism of stabilization of each buffer component with the tested mAb should be studied individually. However, this is beyond the scope of this article.
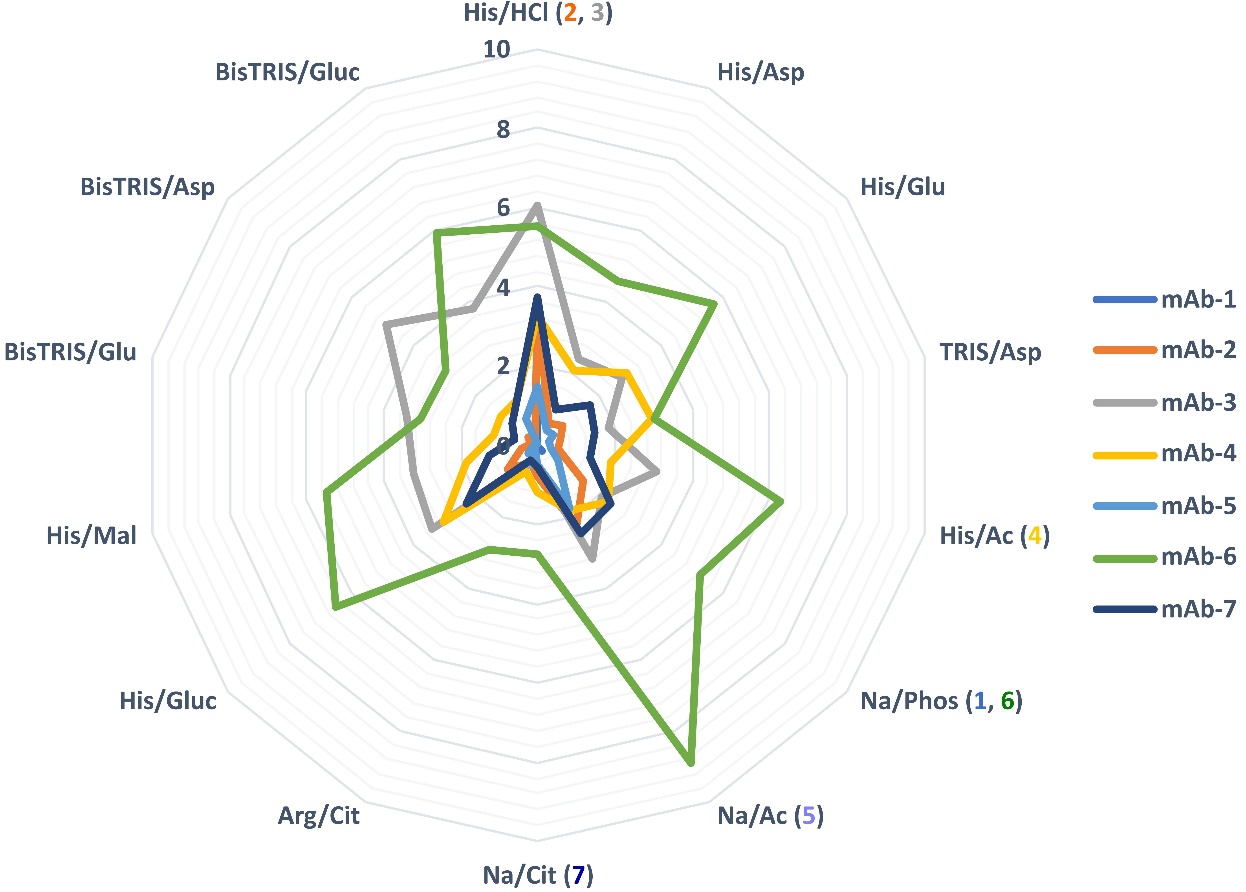
Fig. 2. Growth of aggregates (in %) after 10 freeze-thaw cycles for 7 mAbs at cmAb of 1 mg mL–1 in the buffers listed in Table I. The reference buffer for each mAb is specified in parentheses following the buffer abbreviation. Vertical numbers from 0 to 10 represent the percentage of HMW’s growth.
Temperature stress
After four weeks at 25 °C, minimal aggregate formation was observed for the mAbs in all tested buffers (Table S3). Only two buffers, bis-TRIS/glucuronate and histidine/aspartate, exhibited notable effects on aggregate formation. Bis-TRIS glucuronate resulted in the highest aggregate levels for mAbs 1–4, while histidine/aspartate had a similar effect on mAbs 5 and 6. However, significant aggregate content was only observed for mAbs 1, 5, and 6, while the remaining mAbs exhibited negligible or no aggregate formation. This indicates that the majority of tested alternative buffers successfully stabilize mAb in the formulation.
Similar results were obtained after 12 weeks at 25 °C, with most mAb formulations showing minimal aggregated formation (Table S4). Once again, bis-TRIS/glucuronate stood out, causing the most notable aggregate formation in mAbs 1–6. Overall, all other tested buffers demonstrated negligible or no aggregate formation, reinforcing the conclusion from the previous paragraph.
Furthermore, SEC results after four weeks at 40 °C revealed significant differences between the tested buffer systems, despite overall good stabilization of all mAbs (Table S5). Buffers containing glucuronic acid as a counterion once again exhibited the highest amount of aggregate levels.
Three-component buffer systems with the same MBC concentration at mAb concentration of 1 mg mL–1
Five alternative histidine buffers were tested and are listed in Table II. These buffers contained a third component, with arginine or lysine added at a final concentration of 50 mmol L–1, in addition to the standard counterions (chloride, glutamate, and aspartate ion). All samples had a pH of 6 and underwent stress conditions, including 4 weeks at 25 °C, 12 weeks at 25 °C, 4 weeks at 40 °C, and 10 cycles of freeze-thaw stress. The difference between aggregate content after the stress period and at t0 was calculated. Reference buffers, along with buffers without added counterions, were used for comparison.
Table II. Buffers with a third component used in stability testing of seven mAbs at a mAb concentration of 1 mg mL–1. The MBCs in the first column are marked in bold
Temperature stress
After 4 weeks at 25 °C, negligible amounts of aggregates were observed (Table S6). Only mAb-6 showed notable growth after 12 weeks at 25 °C and 4 weeks at 40 °C (Table S7 and S8). In both cases, the addition of 50 mmol L–1 Lys resulted in increased aggregate formation, while the addition of arginine stabilized the formulation.
Freeze-thaw stress
Significantly more aggregates were formed during freeze-thaw stress (Fig. 3 and Table S9) compared to temperature stress. Lower amounts of aggregates were only detected in the mAb-1 formulations, which had previously shown resistance to the mentioned stress. For six of the seven mAbs tested, the addition of arginine to histidine/glutamate and histidine/aspartate buffers resulted in increased aggregate formation. Interestingly, the addition of arginine or lysine to the histidine/HCl buffer proved to be very effective leading to lower aggregates levels compared to buffers without a third component.
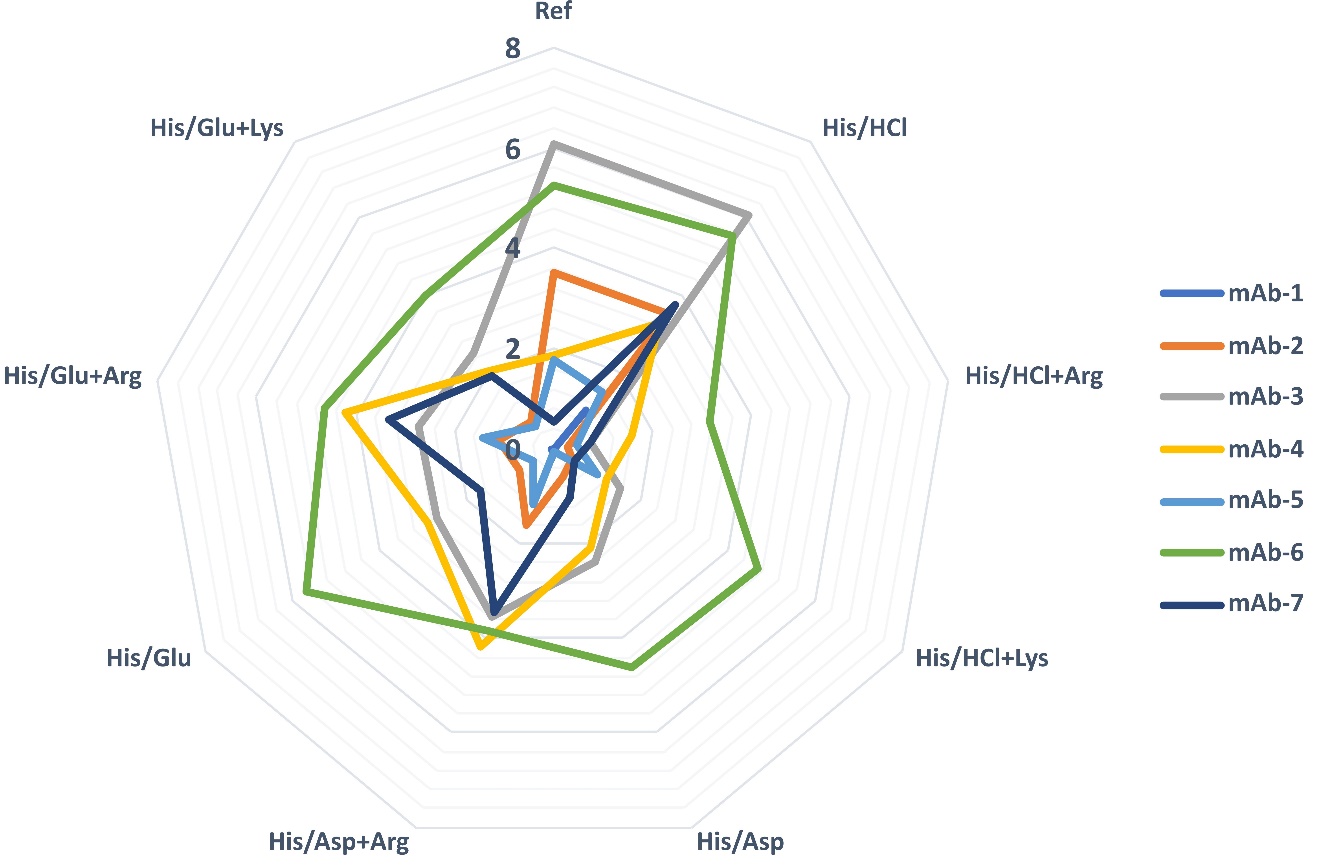
Fig. 3. Growth of aggregates (in %) after 10 freeze-thaw cycles for 7 mAbs in three-component buffers at cmAb of 1 mg mL–1 in the buffers listed in Table II. Four buffers are included for easier comparison of the third component effect. The reference buffer is marked with “Ref”. Vertical numbers from 0 to 8 represent the percentage of HMW's growth.
Reference-like buffers with increasing protein concentration
We conducted a systematic investigation to test our hypothesis regarding the impact of mAb concentrations on aggregate growth under different stress conditions (discussed later in the paper). Specifically, we formulated each mAb in its respective reference buffer at concentrations of 1, 5, 20, and 50 mg mL–1 and subjected them to three temperature stresses (4 weeks at 25 °C, 12 weeks at 25 °C, and 4 weeks at 40 °C) as well as freeze-thaw stress. Results are shown in Fig. 4. Our assumption, that higher mAb concentrations would lead to increased aggregate growth, was validated for the temperature stresses. As expected, aggregate formation increased with higher protein concentration for all mAbs and intensified with the levels of stress. Nevertheless, the aggregate content remained very low for all tested mAbs, except for mAb-6, where it was more pronounced after 4 weeks at 40 °C.
However, we observed deviations from this trend in the case of freeze-thaw stresses, with some mAbs 2–6 displaying higher aggregate growth at lower concentrations. We hypothesize that molecular crowding and excluded volume effects may be beneficial for the protein stability of some mAbs. The aforementioned effects are more pronounced in samples with higher protein concentrations, making unfolding at the ice-liquid interface that forms during the freezing process and the resulting aggregation less likely (17).
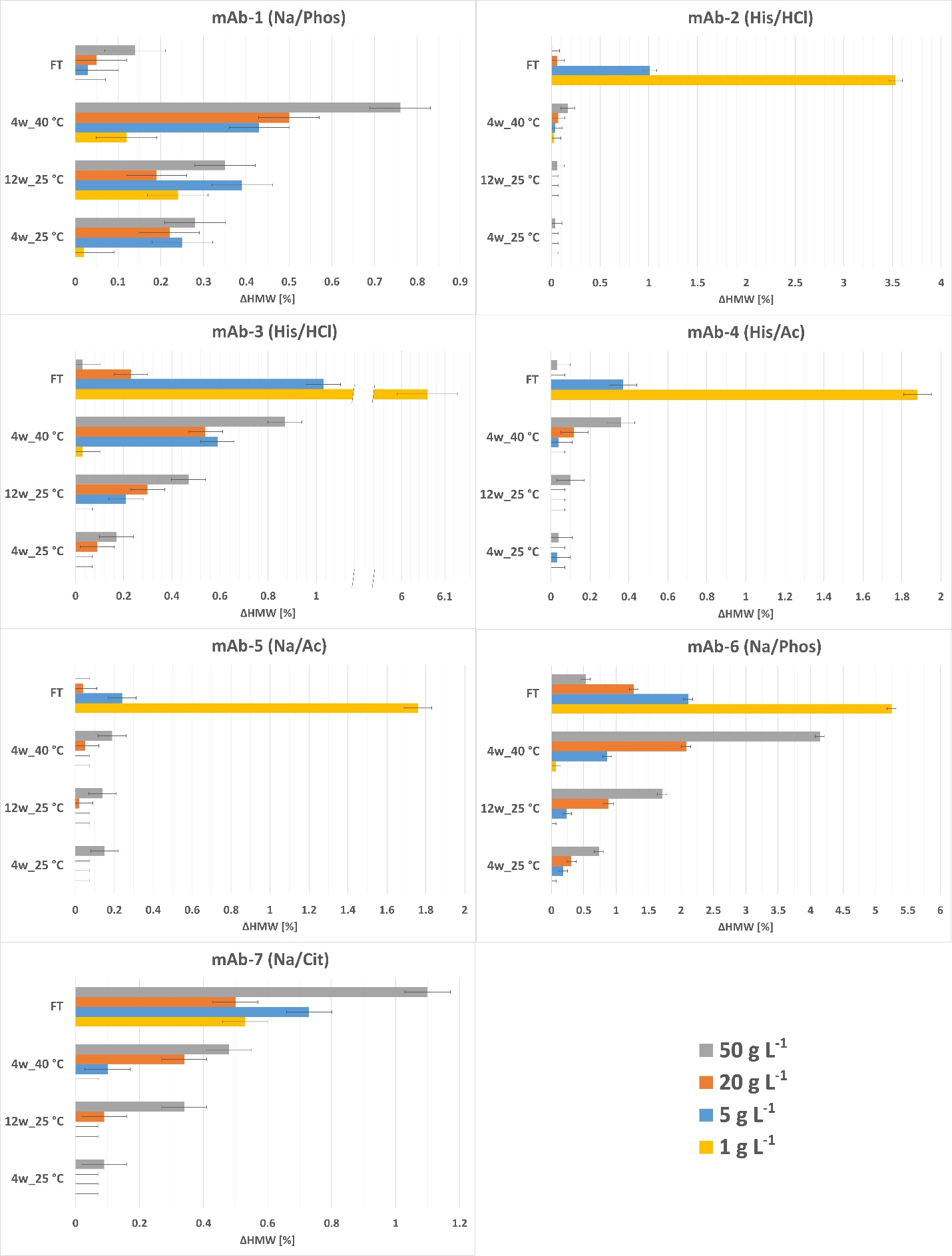
Fig. 4. Growth of aggregates after 10 freeze-thaw cycles, 4 weeks at 25 °C, 12 weeks at 25 °C, and 4 weeks at 40 °C for all seven mAbs at different concentrations in the reference buffer. The legend in the lower right indicates the bar colors corresponding to the mAb concentrations in the graphs. The heading of each graph indicates the reference buffer used for the respective mAb.
Buffer systems with the same ionic strength at a mAb concentration of 50 mg mL–1
For this set of samples, the mAb concentration was increased to 50 mg mL–1. Table III presents a set of buffers again with an ionic strength of 0.028 mol L–1 and pH of 6. Three buffers of interest with different ionic strengths, indicated with an up (ꜛ) or down (ꜜ) arrow in parentheses next to the buffer, were included to examine their effects on stability. The buffers were tested with the same seven mAbs, but were subjected only to 4 weeks at 40 °C. The difference between the aggregate content after the stress period and at t0 was calculated.
Table III. Buffers used in the stability testing of seven mAbs at a concentration of 50 mg mL–1. The MBCs in the first column are marked in bold
Aggregate growth in alternative buffers after four weeks at 40 °C is depicted in Fig. 5. Notably, mAbs 3 and 6 exhibit higher levels of aggregate formation. Interestingly, the majority of tested alternative buffers outperformed the conventionally used Na/phosphate under this type of stress. However, the increased ionic strength of the histidine/malate and the arginine/citrate buffer posed a challenge only for mAb-6. It can be concluded that there are many suitable buffers as alternatives to reference buffers and that ionic strength can be of great importance in some cases and should be chosen carefully.
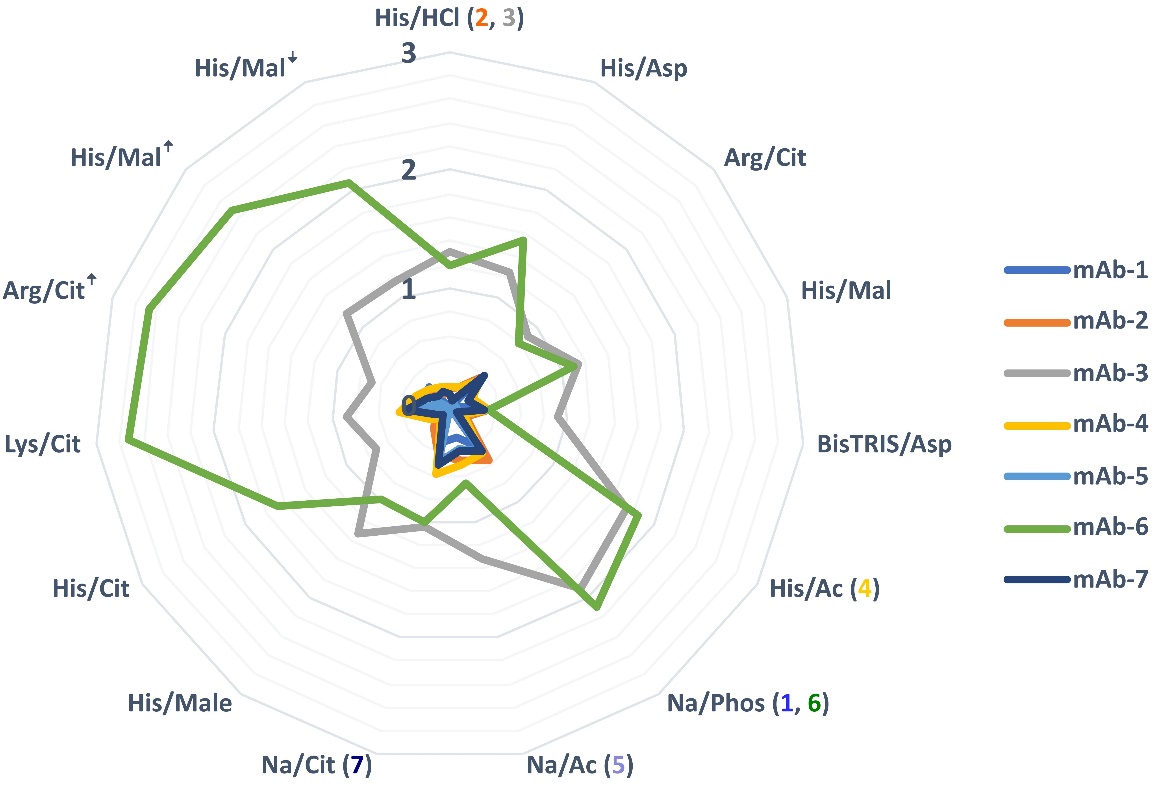
Fig. 5. Growth of aggregates (in %) after four weeks at 40 °C for 7 mAbs in the buffers listed in Table III. The reference buffer for each mAb is specified in parentheses following the buffer abbreviation. Buffers with different ionic strength are marked with an arrow pointing up or down. Vertical numbers from 0 to 3 represent the percentage of HMW's growth.
Highly concentrated formulations – histidine and citrate buffers with various counterions
Lastly, we investigated the performance of four mAbs (3, 5, 6, and 7) in 12 buffers with a consistent MBC concentration of 15 mmol L–1 – histidine and citrate (Table IV). The pH of all samples was maintained at 6, and various counterions were employed. Highly concentrated formulations were prepared with a mAb concentration of 100 mg mL–1, except for mAb-7, which was at 80 mg mL–1 due to initial upconcentration challenges. Separate samples of the formulations were subjected to light stress and 4 weeks at 40 °C. The difference in aggregate content between the stress period and t0 was calculated.
Table IV. Buffers used in stability testing of four mAbs at 100 mg mL–1. MBCs in the first column are marked in bold
Following temperature stress (Fig. 6 Left and Table S10), mAb-5 and mAb-7 still maintained negligible amounts of aggregates. Intriguingly, for mAb-3, citrate-based buffers exhibited superior stabilization compared to histidine buffers, while the opposite was observed for mAb-6. Both mAb-3 and mAb-6 showed excellent stability by hybrid histidine/citrate buffer, further highlighting the significance of counterions in the stabilization process.
Under light stress, substantial aggregate formation was observed in all four mAbs, with mAb-6 reaching up to 50 % of aggregate content (Fig. 6 Right and Table S11). Remarkably, the growth of aggregates was significantly higher (up to two-fold) in non-histidine buffers. We hypothesize that this phenomenon can be attributed to the light-absorbing ability of histidine, which leads to its oxidation and degradation (18, 19). The photosensitivity of histidine has been reported to be greater at pH below 7, which aligns with the conditions of our samples (20). In general, the lysine/citrate buffer stands out for its elevated aggregate formation across all tested buffers, whereas the histidine/phosphate combination emerged as the most effective option.
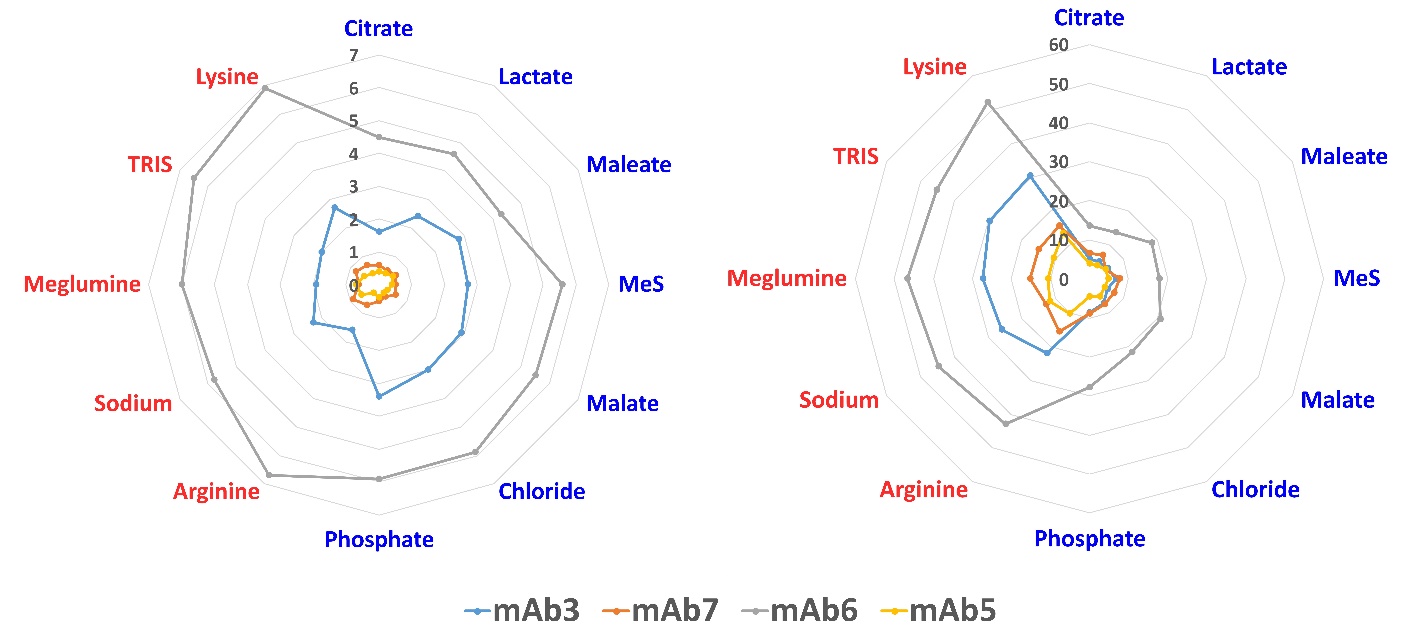
Fig. 6. Summary of aggregates for each counterion with histidine (blue) or citrate (red) as MBC (see Table IV for buffers) after 4 weeks at 40 °C (left) and light stress (right). Each mAb is labeled with a different color (see legend). Vertical numbers from 0 to 7 (left) or 60 (right) represent the percentage of HMW’s growth.
CONCLUSIONS
Numerous alternative buffer systems for biopharmaceutical formulations, that offer comparable or even superior performance in terms of soluble aggregate formation to conventional buffers, have been identified. Temperature, freeze-thaw, and light stress types were employed. Thus, we conclude that the strategy for selecting the optimal buffer in formulation design should not only include conventional buffers in biopharmaceutical formulations (e.g., histidine/HCl, Na/acetate, or Na/phosphate). Many buffer systems with unorthodox counterions and the addition of a third buffer component can stabilize mAb just as well, if not better. It is also critical to identify types of stress, to which mAbs are particularly sensitive, and to use excipients that provide the best stabilization in this case. Given the rapid growth of the biopharmaceutical industry, much more work needs to be put into understanding formulation development, not only to find the right formulation through trial and error but also to explain the stabilization effects of the components and incorporate them into the formulation design.
Funding. – The research was funded by Novartis Pharmaceutical Manufacturing LLC and the Slovenian Research and Innovation Agency core research funding P1-0208.
Conflict of interest. – Blaž Lebar is funded, while Mitja Zidar, Aleš Žula, and Roman Šink are employed by Novartis AG, however, the company had no role in the research, the interpretation, the writing and in the decision to publish. The authors declare no conflict of interest.
Authors contributions. – Formal analysis, B.L., M.Z. and A.Ž.; investigation, B.L.; methodology, B.L. and A.Ž.; visualization, B.L. and J.M.; writing – original draft, B.L; conceptualization, M.Z. and A.Ž.; validation, J.M. and S.P.; writing, review, editing, J.M., A.Ž. and S.P.; project administration, R.Š. and A.Ž.; supervision, R.Š. and A.Ž. All authors have read and agreed to the published version of the manuscript.