Introduction
Heavy metals are naturally occurring elements that have a higher atomic weight and density than water (Tchounwou et al. 2012, Wani et al. 2021). Heavy metal pollution is increasing due to anthropogenic activities, such as mining, smelting, industrial production and metal-containing compounds in household and agriculture (He et al. 2005, Wani et al. 2021). Increasing heavy metal pollution gradually adversely affects the ecosystem, with terrestrial plants being particularly susceptible compared to other organisms. Heavy metals are transported to various plant parts after root uptake and then eventually enter the food chain (Ding et al. 2019). An excess of heavy metals, one of which is mercury (Hg), leads to damage to cells, tissues, and enzymes in organisms.
Mercury is a non-essential element and widely accumulates in an ecosystem as an industrial pollutant. Anthropogenic sources for Hg accumulation in soil include mining, gold smelting, fuel combustion, and the industries involved in manufacturing items such as paint, disinfectants, pharmaceuticals, paper, and antimicrobial drugs (Gontia-Mishra et al. 2016). Hg is one of the most important threats to agricultural areas and causes serious damage to crops at various growth stages. Due to its transitional properties Hg is easily taken up by plants, where it inhibits the root growth and development, and affects the water balance and mineral nutrition (Chen et al. 2015). Hg toxicity leads to a reduction in chlorophyll and carotenoid contents due to the peroxidation of thylakoid membranes (Amooaghaie and Enteshari 2017). Also, it induces lipid peroxidation (LPO) and oxidative damage by generating excessive reactive oxygen species (ROS) such as hydrogen peroxide (H2O2), hydroxide radical (OH−) and superoxide anion radical (O2 .-) within plant subcellular structures (Zhou et al. 2007, Gao et al. 2010, Sahu et al. 2012).
Nitric oxide (NO), which is a bioactive gaseous molecule and acts as a signaling molecule, may enhance the plant's response to environmental stresses, including heavy metals, by regulating their physiological and biochemical processes (García-Mata and Lamattina 2013). Additionally, NO acts as an antioxidant, effectively preventing and scavenging ROS in cells, thus alleviating oxidative damage (Chen et al. 2018). There are some previous studies about the role of exogenous NO in plants under heavy metal stress. They reported that the application of NO donors not only increased chlorophyll content, biomass and root length but it also decreased lipid peroxidation and ROS production under heavy metal stress such as cadmium (Cd), copper (Cu), nickel (Ni), zinc (Zn), and arsenic (As) (Terrón-Camero et al. 2019). Furthermore, NO may decrease Cu, Cd, lead (Pb) and Pb/Cd-induced oxidative damage by restraining heavy metal uptake capacity of plant root system in ryegrass, peanut, rice and alfalfa (Mostofa et al. 2014, Bai et al. 2015, Dong et al. 2016, Fang et al. 2019). Moreover, NO could alleviate phytotoxicity by directly regulating accumulation and translocation of mercury in rice (Chen et al. 2015). The detoxification by NO might be related to the modulation of cell wall components, pectin and hemicelluloses (Chen et al. 2015). However, there is little information regarding the role of NO in regulating Hg-induced stress in maize.
Maize ( Zea mays L.), one of the oldest field crops cultivated by humans, is a vital cereal plant from the Poaceae family, widely grown worldwide. Due to its rich nutritional content, maize is very valuable for both human and animal nutrition, with a wide range of uses. Starch, glucose and corn oil obtained from corn grain, which are of great importance for the sufficient and economic production of plant-based proteins in the world, are used as raw materials in the economy. The protection of maize under adverse conditions is important in terms of both food supply and human health.
Based on the above studies, we hypothesized that NO is quite crucial in helping plant tolerance under Hg stress. Therefore, our aim was to show that NO is able to alleviate Hg-induced toxicity by activating the antioxidant system and reducing oxidative stress in maize seedlings.
Materials and methods
Plant material and growing conditions
In this study we utilized maize ( Zea mays L. cv. Arifiye-2) as plant material, sodium nitroprusside (SNP) as the source of nitric oxide (NO), and mercury chloride (HgCl2) as the source of mercury (Hg). Maize seeds were subjected to a surface sterilization in 70% ethanol for 1 min, subsequently in 5% sodium hypochlorite for 5 min, and then rinsed 6 times with distilled water.
In preliminary experiments surface-sterilized maize seeds were sown on Petri dishes (15 cm diameter) with double-layer filter paper and treated with different HgCl2 concentrations (0, 1, 5, 10, 20, 40, 50, 100, 200, 500 and 1000 µM) for 5 days in a growth chamber at 25 ± 2 °C. The 100 µM HgCl2 was chosen for further experiments because it caused at least a 50% reduction in germination rate and root length. A similar study was conducted to determine the appropriate SNP concentration by sowing maize seeds on Petri dishes with 100 µM HgCl2 and different SNP concentrations, and 0.1 µM SNP was chosen because it markedly alleviated negative growth effects of Hg.
For treatments with 100 µM HgCl2 and 0.1 µM SNP, the plants were grown in a hydroponic system containing 1/2 diluted Hoagland solution (Hoagland and Arnon 1950) in conditions of 16 h day length, 25/20 °C (day/night), and 60% relative humidity. When seedlings were 8-days old, initial treatment with 0.1 µM SNP was applied to the hydroponic medium. Half-strength Hoagland’s solution used as control. After 24 h, the seedlings were exposed to 100 µM HgCl2 and then seedlings were harvested after 3 days of Hg treatment. These periods (24 h and 3 days) were determined according to a previous experiment and literature (Chen et al. 2015). Harvested seedlings were stored at -80 °C for subsequent experiments or immediately used for measurement of growth parameters. The purity of all chemicals was more than 98%.
Measurement of seedling growth and photosynthetic pigments content
Ten randomly selected seedlings were used for morphological measurements. Firstly, fresh weight (FW) and shoot length (SL) were measured using a balance and a scale. Then the seedlings were put in an oven for 72 h at 80 °C to detect dry weight (DW). The chlorophyll pigments content in the leaves was detected in acetone extracts by the spectrophotometric method according to Lichtenthaler (1987) and was expressed as mg g-1 fresh weight (FW).
Determination of reactive oxygen species, lipid peroxidation and electrolyte leakage
Hydrogen peroxide (H2O2) level was determined according to the method of Hu et al. (2005). Briefly, 0.5 g of the samples were homogenized in 10 mL of cold acetone and then centrifuged at 5000 × g for 15 min at + 4 °C. Then, 0.5 mL of the supernatant was combined with 0.15 mL of 5% Ti(SO4)2 and 0.3 mL of 19% NH4OH. The mixture was centrifuged at 3000 × g for 10 min at + 4 °C. The pellet was washed twice with cold acetone and dissolved in 3 mL of 1 M H2SO4. After filtration, absorbance measurement of filtrate was measured at 415 nm using H2SO4 as a blank. The amount of H2O2 was calculated by a constructed standard curve and defined as µg g-1 fresh weight (FW).
The production of super oxide anion (O2 .-) was determined by Liu et al. (2007). Fresh tissue (0.5 g) was homogenized in 3 mL of 50 mM phosphate buffer (pH 7.8), and the homogenate was centrifuged at 10000 × g for 10 min. The supernatant (0.1 mL) was combined with 0.9 mL of 65 mM phosphate buffer (pH 7.8) and 0.1 mL of 10 mM hydroxylamine hydrochloride. It was incubated at 25 °C for 15 min, and afterwards 1 mL of the mixture, 1 mL of 17 mM 1-naphthylamine and 1 mL of 17 mM anhydrous amino benzene sulfonic acid were combined and again incubated at 25 °C for 20 min. Butyl alcohol (3 mL) was supplemented to the mixture and the absorbance was measured at 530 nm. NaNO2 was used for a standard curve to calculate the content of O2.- which was defined as µg g-1 fresh weight (FW).
The lipid peroxidation (LPO) level was determined by measuring the content of malondialdehyde (MDA). Briefly, 0.5 g of the samples were homogenized in 5 mL of 1% trichloroacetic acid (TCA) and then centrifuged at 12000 × g for 20 min. One mL of the supernatant was combined with 4 mL of 0.5% TBA (2-thiobarbituric acid) in 20% TCA. The reaction mixture was incubated for 30 min in a boiling water bath, and then the reaction was stopped in an ice bath. Then the reaction tubes were centrifuged again at 5000 × g for 10 min. The absorbance of the supernatant was measured at 532 nm and it was corrected by subtracting nonspecific absorbance at 600 nm. MDA level was calculated using extinction coefficient of 155 mM-1 cm-1 and expressed as nmol g-1 fresh weight (FW) (Heath and Packer 1968).
For electrolyte leakage (EL), the fresh leaves (0.1 g) washed with distilled water were placed in each of the test tubes including 4 mL distilled water and incubated for 4 hours at + 4 °C. Then, the amount of ions that passed into the pure water in the tubes was measured by an electrical conductivity meter (Griffith et al. 1992).
Activity of antioxidant enzymes and their isoenzyme profiles
The sample (0.2 g) was ground in 2 mL of extraction buffer (0.1 M KH2PO4, pH 7.0) including 0.2% polyvinylpyrrolidone and 1 mM ethylenediaminetetraacetic acid (EDTA), and the homogenate was centrifuged at 12000 × g for 15 min at + 4 °C. The supernatant was collected for enzyme activity and also used for protein determination by using bicinchoninic acid assay (BCA) reagent spectrophotometrically at 562 nm (Smith et al. 1985).
Activity of antioxidant enzymes was measured according to the method of Agarwal and Pandey (2004). Superoxide dismutase (SOD, EC 1.15.1.1) activity was described as one unit (U), the value of enzyme that inhibited 50% of the photoreduction of nitroblue tetrazolium chloride (NBT). Activity of SOD enzyme was recorded as U mg-1 protein. Peroxidase activity (POX, EC 1.11.1.7) was assayed by determining the absorbance increase at 470 nm caused by tetraguaiacol, which is a product of the reaction in which guaiacol and H2O2 are used as substrates. One unit of POX is identified as the value of enzyme that increases the absorbance at a rate of 0.01 within 1 min at 25 °C, and data are recorded as U mg-1 protein. Catalase (CAT, EC 1.11. 1.6) activity is based on the measurement of the decrease in absorbance at 240 nm when CAT provides the conversion of H2O2 to O2 and H2O. One unit of CAT is determined as the value of enzyme disrupting 1 mM H2O2 within 1 min at 25 °C, and data are expressed as U mg-1 protein. Glutathione reductase (GR, EC 1.6.4.2) activity was measured by monitoring glutathione dependent oxidation of NADPH at 340 nm. The reaction mixture included 0.2 mM NADPH, 1 mM EDTA, 3 mM MgCl2, 0.5 mM oxidized glutathione (GSSG), and 100 mM Tris-HCl (pH 7.8). Data were expressed as U mg-1 protein.
Native proteins were run on polyacrylamide gel electrophoresis (PAGE) under non-denaturing conditions as suggested by Laemmli (1970). For SOD isoenzymes, the gel was incubated in 0.05 M KH2PO4 (pH 7.8) containing 0.24 mM NBT, 33.2 μM riboflavin, 0.2% N,N,N′,N′ -tetramethylethylenediamine (TEMED), and 1 mM EDTA on a shaker in the dark for 30 min at 37 °C. Then, the gel was placed in 0.05 M potassium phosphate buffer (pH 7.8) containing 1 mM EDTA and incubated under white fluorescent light for 10 – 30 min to determine isoenzymes (Beauchamp and Fridovich 1971). POX and CAT isoenzymes were monitored according to Weydert and Cullen (2010). For POX isoenzymes, the activity staining was realized after incubation for 30 min in 0.2 M sodium acetate buffer (pH 5.0) containing 30 mM H2O2 and 10 mM guaiacol. For CAT isoenzymes, the gel was incubated in 30 mM H2O2 for 10 min, it was stained with 2% FeCl3 and 2% K3FeCN6 solutions. GR staining was carried out by incubation in a reaction solution including 50 mL of Tris-HC1 (pH 7.5) containing 10 mg of 3-(4, 5-dimethylthiazol-24)-2,5-diphenyl tetrazolium bromide, 10 mg of 2,6-dichlorophenolindophenol, 3.4 mM GSSG, and 0.5 mM NADPH. Duplicate gels were assayed for GR activity, one with and one without GSSG (Rao et al. 1996).
Determination of glutathione, ascorbic acid, and proline contents
Reduced glutathione (GSH) content was determined enzymatically using the method of Griffith (1980) with slight modification. Fresh tissue (0.2 g) was homogenized in 2 mL of 5% meta-phosphoric acid and centrifuged at 12000 × g for 20 min at 4 °C. The reaction mixture consisted of 150 µL of the supernatant and 1850 µL of KH2PO4 (50 mM, pH 7.5) including 2.5 mM EDTA, 1 mM 5,5′-dithiobis-(2-nitrobenzoic acid) (DTNB), 0.3 U glutathione reductase, and 1 mM NADPH. The increase in absorbance at 412 nm was monitored for 3 min at 25 °C. The amount of GSH was calculated by a constructed standard curve and data were expressed as nmol g-1 fresh weight (FW).
The content of ascorbate (AsA) was determined as described by Okamura (1980). Fresh tissue (0.2 g) taken from the powder obtained from liquid nitrogen grinding was homogenized in 2 mL of 5 % TCA. The extract was centrifuged at 12000 × g for 10 min at 4 °C. For AsA content, reaction mixture consisted of 1 mL of supernatant and 1.5 mL of KH2PO4 (pH 7.4) including 10 mM dithiothreitol (DTT), 0.014% N-ethylmaleimide, 2.6% TCA, 11.7% H3PO4, 1% 2,2′-dipyridyl, and 0.3% FeCl3. Samples were incubated for 60 min at 37 °C and the absorbance was recorded spectrophotometrically at 525 nm. The amount of AsA was calculated by a constructed standard curve and data were expressed as nmol g-1 fresh weight (FW).
Colorimetric detection of proline was determined based on proline’s reaction with ninhydrin (Bates et al., 1973). The amount of proline was calculated by a constructed standard curve and data were expressed as µg g-1 fresh weight (FW).
Determination of nutrient element contents
The seedlings (0.5) were powdered in a mill and sieved (0.5 mm) and dried at 70 °C and digested with concentrated HNO3 in a microwave system CEM, Mars 5 (CEM Corp., USA). In the seedlings digested, the concentrations of Na, Ca, Mn, and K were measured by inductively coupled plasma-mass spectrometry (ICP-MS, Agilent-7800, Agilent Technologies, Tokyo, Japan).
Statistical analysis
Each experiment was repeated at least three times in three replicates. Analysis of variance (ANOVA) was conducted in a one-way ANOVA test using SPSS 17.0 for Microsoft Windows, and means were compared by Duncan test at the 0.05 level of confidence. The data were represented as the mean ± standard error from three experiments.
Results
Physiological growth parameters
The outcomes of the fresh weight (FW), the dry weight (DW), and the shoot length (SL) for the control groups (control and SNP) and mercury (Hg) treated groups (Hg and SNP + Hg) are presented in Tab. 1. Exposure to toxic Hg caused a significant inhibition (P < 0.05) by 36% in FW, 20% in DW, and 18.8% in SL compared to the control (Tab. 1). But, when the SNP (as a donor of NO) was applied to the seedlings exposed to Hg toxicity (SNP + Hg), the same parameters were increased by 24%, 20%, and 6%, respectively, in comparison to Hg application alone (Tab. 1).
Tab. 1. Effects of SNP (as a donor of NO) and Hg on fresh and dry weight, and height of maize seedlings. Treatments: Control − half-strength Hoagland’s solution, Hg − 100 µM HgCl2, SNP + Hg − 0.1 µM SNP + 100 µM HgCl2, SNP − 0.1 µM SNP. Values are means of three independent experiments ± standard errors. Different letters in the same column indicate statistically significant differences between means (P < 0.05).
Chlorophyll content
Hg exposure resulted in decreased chlorophyll content in maize seedlings (Tab. 2), with reductions of 9% in chlorophyll-a (Chl-a), 6% in chlorophyll-b (Chl-b), and 7.79% in total chlorophyll content compared to the control (Tab. 2). Moreover, the inhibitory effect of Hg on Chl-a content was more pronounced than on Chl-b. However, the application of NO treatment (SNP + Hg) to Hg-exposed plants led to significant increases of 12.7% in Chl-a, 28% in Chl-b, and 19.45% in total chlorophyll content compared to control plants (Tab. 2). Remarkably, the SNP treatment seemed to have a greater impact on Chl-b content in Hg-exposed seedlings. Interestingly, SNP alone led to a decrease in chlorophyll content compared to control plants (Tab. 2).
Tab. 2. Changes in the content of chlorophyll-a (Chl-a), chlorophyll-b (Chl-b), and total chlorophyll (total chl) of maize seedlings under Hg treatment with or without SNP (as a donor of NO). Treatments: Control − half-strength Hoagland’s solution, Hg − 100 µM HgCl2, SNP + Hg − 0.1 µM SNP + 100 µM HgCl2, SNP − 0.1 µM SNP. Values are means of three independent experiments ± standard errors. Different letters in the same column indicate statistically significant differences between means (P < 0.05).
Reactive oxygen species, lipid peroxidation and electrolyte leakage
Table 2 displays the outcomes of ROS such as H2O2 and O2 .-, lipid peroxidation (MDA) and electrolyte leakage (EL) measurements for the control groups and the Hg-treated groups. NO treatment under normal conditions did not have any effect (P > 0.05) on H2O2 and O2 .- contents. Conversely, exposure to Hg increased H2O2 content by 44% and the O2 .- content by 8% compared to the control (Tab. 3).
Tab. 3. Effects of SNP (as a donor of NO) and Hg on reactive oxygen species (H2O2 and O2 .-), lipid peroxidation (MDA) and electrolyte leakage (EL) levels of maize seedlings. Treatments: Control − half-strength Hoagland’s solution, Hg − 100 µM HgCl2, SNP + Hg − 0.1 µM SNP + 100 µM HgCl2, SNP − 0.1 µM SNP. Values are means of three independent experiments ± standard errors. Different letters in the same column indicate statistically significant differences between means (P < 0.05).
However, in the SNP + Hg seedlings, there was a significant decrease in generation of H2O2 and O2 .- by 21% and 11%, respectively (Tab. 3). Moreover, toxic Hg increased MDA content by 47% and EL level by 408% indicating oxidative damage to membranes but SNP + Hg significantly reduced MDA content by 15.6% and EL level by 37.6% (Tab. 3).
Activities and isozyme profiles of antioxidant enzymes
Figure 1 and Figure 2 present the activities and isoenzyme profiles of antioxidant enzymes, including superoxide dismutase (SOD), catalase (CAT), peroxidase (POX), and glutathione reductase (GR). Exposure to Hg led to reductions in the activities of SOD, CAT, POX, and GR by 11.6%, 9.4%, 13.9%, and 19.5%, respectively. Interestingly, the addition of SNP + Hg significantly stimulated the activities of these enzymes by 9.5%, 20.6%, 31.9%, and 15.2%, respectively (Fig. 1).
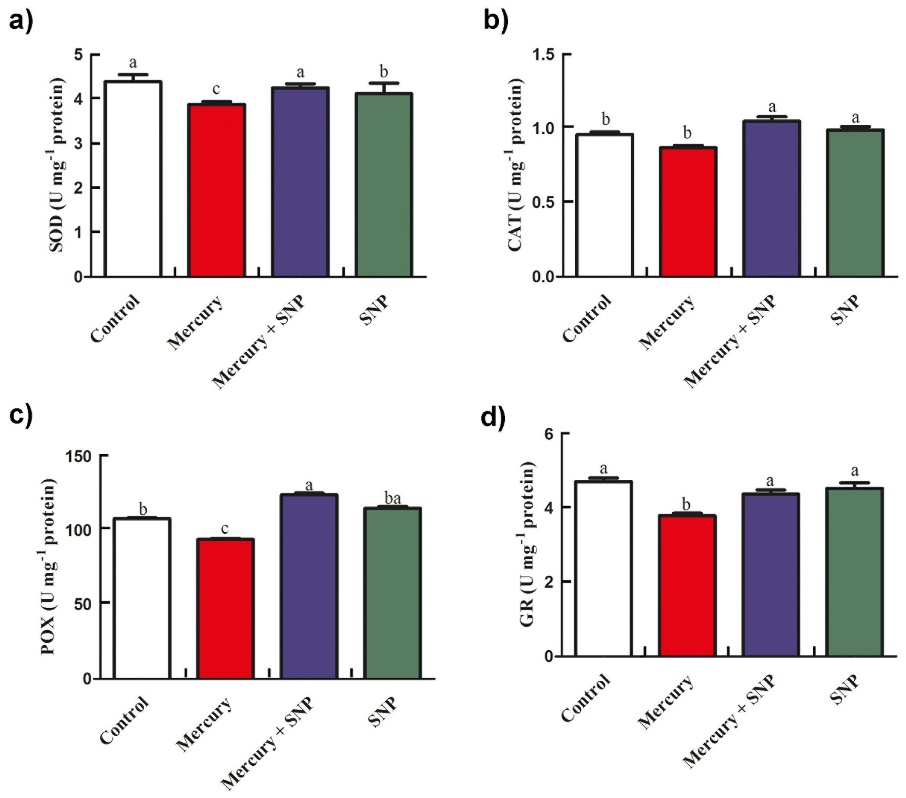
Fig. 1. Effects of SNP (as a donor of NO) and Hg on activities of antioxidant enzymes in maize seedlings. a) Superoxide dismutase (SOD) b) Catalase (CAT) c) Peroxidase (POX) d) Glutathione reductase (GR). Treatments: Control − half-strength Hoagland’s solution, Hg − 100 µM HgCl2, SNP + Hg − 0.1 µM SNP + 100 µM HgCl2, SNP − 0.1 µM SNP. Values are means of three independent experiments; bars indicate standard errors. Different letters show significant differences (P < 0.05) between maize groups.
The isoenzyme profile analysis, depicted in Fig. 2, revealed distinct bands for SOD (SOD 1-5), CAT (CAT 1-2), POX (POX 1-4), and GR (GR 1-2). Notably, exposure to Hg resulted in increased band densities for some isoenzymes of SOD, POD, and GR compared to the control. Furthermore, some isoenzymes appear thicker in maize treated with SNP + Hg, indicating a potential enhancement of the enzyme activities.
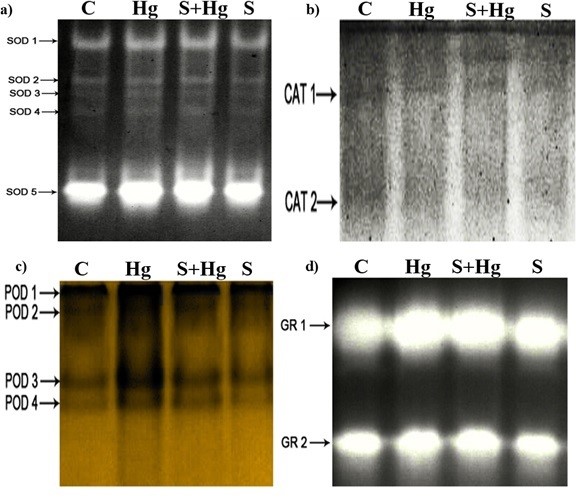
Fig. 2. Effects of SNP (as a donor of NO) and Hg on isoenzyme profiles of maize seedlings a) Superoxide dismutase (SOD), b) Catalase (CAT), c) Peroxidase (POX), d) Glutathione reductase (GR). Treatments: Control − half-strength Hoagland’s solution, Hg − 100 µM HgCl2, S + Hg − 0.1 µM SNP + 100 µM HgCl2, S − 0.1 µM SNP.
AsA, GSH, and proline contents
Exposure to Hg significantly elevated the contents of glutathione (GSH) by 21.5%, ascorbate (AsA) by 22.5%, and proline by 31.1% compared to control plants (Tab. 4).
Tab. 4. Effects of SNP (as a donor of NO) and Hg on glutathione (GSH), ascorbate (AsA) and proline contents of maize seedlings. Treatments: Control − half-strength Hoagland’s solution, Hg − 100 µM HgCl2, SNP + Hg − 0.1 µM SNP + 100 µM HgCl2, SNP − 0.1 µM SNP. Values are means of three independent experiments ± standard errors. Different letters in the same column indicate statistically significant differences between means (P < 0.05).
Notably, SNP + Hg treatment also increased the contents of AsA (11.4%) and proline (26.4%), although GSH levels remained unchanged (Tab. 4). These findings indicate the participation of NO in augmenting non-enzymatic antioxidant defenses.
Some nutrient element contents
Exposure to toxic Hg increased sodium (Na) content by 29% compared to the control plants but reduced the content of manganese (Mn) and calcium (Ca) by 39.4% and 13.3%, respectively. The potassium (K) content, however, remained unchanged (Tab. 5). Intriguingly, treatment with NO increased Mn and K content by 7.3% and 8.7%, respectively, while reducing Na content by 26% in maize seedlings exposed to Hg compared to control plants (Tab. 5). SNP alone increased the amount of K, Ca, Na and Mn in maize seedlings compared to control plants. These findings highlight NO's potential in modulating essential element balances.
Tab. 5. Effects of SNP (as a donor of NO) and Hg on contents of elements of some nutrients of maize seedlings. Treatments: Control − half-strength Hoagland’s solution, Hg − 100 µM HgCl2, SNP + Hg − 0.1 µM SNP + 100 µM HgCl2, SNP − 0.1 µM SNP. Values are means of three independent experiments ± standard errors. Different letters in the same column indicate statistically significant differences between means (P < 0.05). LOD − limit of detection, LOQ − limit of quantitation.
Discussion
Mercury (Hg), a heavy metal known for its high toxicity despite being non-essential, poses a global threat as it is readily absorbed by plants and accumulates across various plant tissues, jeopardizing crop yield and food safety (Wani et al. 2021). The imperative to counteract heavy metal-induced phytotoxicity has driven the search for effective, safe, and economically feasible solutions. Many studies have been carried out on plant signaling molecules that regulate antioxidant defense mechanisms in plants to increase their tolerance to stressful environments including heavy metals such as As, Cd, Al, Hg (Chen et al. 2015, Ahmad et al. 2021, Wani et al. 2021). Especially, previous studies have demonstrated the potential of salicylic acid and carbon monoxide to alleviate Hg toxicity (Zhou et al. 2007), while other research has unveiled the detoxification effects of nitric oxide (NO) on heavy metals like As, Cd, Al, and Ni (Singh et al. 2009, Saxena and Shekhawat 2013). The present study investigated the effects of NO treatment on maize seedlings exposed to Hg toxicity. The results revealed significant alterations in various physiological parameters, ROS levels, antioxidant enzyme activities, antioxidant molecules contents, chlorophyll content, and elemental composition.
The negative impact of toxic Hg on the growth of maize seedlings was evident from the reduction in fresh weight, dry weight, and shoot length. These findings are in agreement with earlier studies that reported the inhibitory effects of heavy metal stress on plant growth (Zhou et al. 2007, Hasanuzzaman et al. 2011). Furthermore, we observed that Hg toxicity in maize induced the generation of excess ROS such as H2O2 and O2.- along with an increase in lipid peroxidation and electrolyte leakage. These outcomes reflect the oxidative stress imposed by Hg on the seedlings, thus leading to cellular damage and inhibition of growth (Gao et al. 2010, Sahu et al. 2012, Chen et al. 2015). Similar findings have been reported in studies focusing on heavy metal-induced oxidative stress (Zhou et al. 2007, D’Souza Myrene and Devaraj 2013). The possible reason for increased ROS despite the observed increase in content of antioxidants (ascorbic acid, glutathione, and proline), could be the reduced activities of key antioxidant enzymes, including SOD, CAT, POX and GR, which we noticed after exposure to Hg. Prior studies have documented heavy metal-induced suppression of antioxidant enzymes (Chen et al. 2018).
The application of NO donor SNP to Hg-exposed maize seedlings led to a significant improvement in FW, DW, and SL. This finding aligns with previous research where exogenous NO application was shown to enhance plant growth and development under stress conditions (Kopyra and Gwóźdź 2003). Moreover, Chen et al. (2015) showed that excessive Hg-induced root growth inhibition and oxidative stress in rice plants can be effectively mitigated through application of NO released from sodium nitroprusside (SNP). NO at low concentrations is a second messenger in plants. It prompts the plant response system into an unfavorable situation. Previous research has demonstrated the antioxidant properties of NO and its ability to attenuate oxidative stress (Kopyra and Gwóźdź 2003, Singh et al. 2009, Kazemi et al. 2010, Cui et al. 2010). In the present study, the application of NO significantly reduced the levels of H2O2 and O2.- in SNP + Hg-treated seedlings, confirming its role in ROS scavenging. Additionally, NO treatment attenuated MDA content and EL level, signifying its protective effect against Hg-induced lipid peroxidation and membrane damage and resulting in improved growth. Remarkably, SNP treatment provided enhanced antioxidant enzyme activities. Isozyme analysis revealed alterations in the density and thickness of bands under Hg stress and SNP treatment. This might signify changes in enzyme isoforms or post-translational modifications in response to NO treatment, indicating a potential regulatory role of NO in the antioxidant defense system (Ahmad et al. 2021). Moreover, SNP treatment further enhanced the contents of AsA and proline indicating its involvement in strengthening the adaptive mechanisms that can counteract Hg-induced oxidative stress as has been already reported in plants under As and Cd toxicity (Hsu and Kao 2004). These observations are in line with the results of Ahmad et al. (2021) showing that the correlation between SNP application and the mitigation of Hg toxicity in soybean cultivars is reinforced by an enhanced antioxidant response and improved AsA-GSH cycle (Ahmad et al. 2021). It is also possible that NO may have acted as an antioxidant molecule as NO produces peroxynitrite, less harmful oxidants, by reacting with O2.- (Saxena and Shekhawat 2013, Chen et al. 2015).
One of the most used methods to understand the effects of abiotic stress factors on plants is to determine chlorophyll (Chl) content (Kupper et al. 1996). Hg exposure led to a reduction in chlorophyll content in maize seedlings, which is consistent with previous reports of heavy metal-induced chlorophyll degradation (Cho and Park 2000, Amooaghaie and Enteshari 2017). Specifically, Chl-a was more adversely affected than Chl-b. This reduction in chlorophyll content indicates impaired photosynthetic capacity, which is crucial for plant growth and development. Strikingly, the addition of NO through SNP treatment reversed this trend, resulting in a significant increase in Chl-a, Chl-b, and total chlorophyll content in SNP + Hg-treated plants. These findings suggest that NO treatment can mitigate Hg-induced chlorophyll degradation, potentially by maintaining chlorophyll synthesis or preventing its breakdown.
The disbalance in plant nutrients may disrupt ion homeostasis and interfere with various metabolic processes. The altered elemental composition under Hg stress, including increased sodium (Na) and reduced manganese (Mn) and calcium (Ca) contents, corroborates with established findings on heavy metal-induced disruptions in mineral uptake (Fang et al. 2019). NO treatment led to a rebalancing effect by decreasing Na and enhancing Mn and potassium (K) contents. Nutrient elements such as Mn, K and Ca have an important role as cofactors in cells and it is essential that under stress conditions the cytosol maintain a high K+/Na+ ratio for optimal metabolic functions. NO may protect the maize seedlings against toxic Hg by increasing the K+/Na+ ratio as increase of Na+ in cells disrupts the function of many proteins. This suggests a potential role of NO in modulating ion uptake, transport mechanisms and ion homeostasis, a critical factor in stress tolerance (García-Mata and Lamattina 2013).
In conclusion, the findings of this study highlight the protective role of exogenous NO (SNP) against mercury (Hg) toxicity in maize seedlings. The observed improvements in physiological parameters, attenuation of oxidative stress, and restoration of antioxidant enzyme activities, modulation of non-enzymatic antioxidants, chlorophyll preservation, and essential element balance suggest NO as a valuable candidate for enhancing plant resilience against heavy metal toxicity.