Introduction
Abiotic stress factors affect the development, survival, biomass production, and yield of plants. Among the abiotic stress factors, heat disrupts plants by damaging their metabolism, in various ways. However, plants have developed different mechanisms to tolerate or avoid high temperatures. High-temperature tolerance is defined as the capacity of the plant to grow under high temperatures and still be economically productive (Sezgin Muslu and Kadıoğlu 2021). Heliotropium thermophilum (Boraginaceae), a heat-tolerant plant species, is an endemic and thermophilic flowering plant. The natural distribution area of the plant is a geothermal region in Buharkent, Aydın, Türkiye with an optimum soil temperature range, between 55 and 65 °C. In addition, there is a high concentration of sulfate anion (772 mg L-1) in the soil environment where the plant grows, which is caused by the oxidation of H2S gas escaping from magma (Tan et al. 2008). It is widely acknowledged that in these conditions the plants attain their ideal nutrient status for optimal productivity and tolerance to environmental constraints.
The sulfur content in plant cells varies with plant growth and developmental stage and the length and intensity of exposure to stress conditions (Ihsan et al. 2019). Sulfur is found in the composition of important biomolecules, including specific vitamins like thiamine and biotin, amino acids such as cysteine and methionine, antioxidants like glutathione (GSH), thioredoxin, fatty acid, i.e., lipoic acid, and glucosinolate (Ali et al. 2019). It has been established that all these sulfur-containing biomolecules have a role in detoxifying reactive oxygen species (ROS) and redox control of proteins under stress conditions (Hill et al. 2022), but are also important signal compounds in plants (Narayan et. al 2023). During the stress conditions, such as heat and drought, GSH undergoes oxidation to glutathione disulfide (GSSG), which is essential for maintaining the optimal functioning of cellular and biochemical redox systems within plant cells (Hasanuzzaman et al. 2017). In addition, GSH is involved in the cellular redox buffer system and acts as an electron donor to remove ROS which may appear in plant metabolic pathways as a result of oxidative stress due to elevated temperatures (Dard et al. 2023). Methionine plays an important role in the nutrition of plants and the translation of mRNAs (Hesse et al. 2004), but is also involved in ROS detoxification in the response to high temperatures (Ihsan et al. 2019). For example, a chloroplast low molecular weight heat shock protein (HSP), which is rich in methionine, plays a crucial role in safeguarding the transportation of electrons from photosystem II when a plant is subjected to thermal stress (Heckathorn et al. 1998). Additionally, chloroplastic HSPs rich in methionine play a direct role in the thermotolerance of the photosynthetic machinery in plants (Sehar et al. 2022).
Heat shock transcription factors (HSF) also play important roles in regulating plant thermotolerance. The rapid synthesis and accumulation of HSPs, which are molecular chaperones that prevent protein aggregation and maintain cellular protein homeostasis, are regulated by HSFs (Kang et al. 2022). For example, an enhanced ability to withstand high temperatures has been observed in wheat plants that overexpress the TaHSFA6f gene that regulates a suite of heat stress protection genes in wheat, including TaHsps (TaHSP16.8, TaHSP17, TaHSP17.3, and TaHSP90.1-A1), TaRof1, galactinol synthase, and glutathione-S-transferase (Xue et al. 2015) Moreover, when soil temperature rose from 20 to 80 °C, the expression levels of HSFA4A, HSFA3 and HSF4 were significantly upregulated in H. thermophilum (Sezgin Muslu and Kadıoğlu, 2021). However, there is no information in the relevant literature cited about how HSFs are affected by sulfur content in plants at high temperatures.
In our study, we hypothesized that there may be a link between the high sulfate concentration in the environment where H. thermophilum grows and its resilience to high soil temperatures. To test the hypothesis and clarify the possible role of sulfur in high temperature tolerance, H. thermophilum plants were grown in sulfur-deficient growth media with two different sulfate levels. Then, the changes in osmotic balance, antioxidant system, and HSF expressions during high temperature as a result of sulfur deficiency were studied.
Material and methods
Plant growth, sulfur deficiency, and temperature applications
Seeds of H. thermophilum, were exposed to surface sterilization with 0.1% hypochloric acid for 5 min in a laminar flow cabinet, then 50 µM GA3 was applied at +4 ºC for two days to break seed dormancy. The seeds were sown in vermiculite containing two low-sulfate media (0.15 and 0.30 mM SO42−) or full nutrition (FN) medium (control), prepared based on the Hoagland solution recipe (On-line Suppl. Tab. 1) (Bielecka, 2007). MgCl2 was used instead of MgSO4 to create sulfur deficiency. Seeds were sown in plastic trays including vermiculite (8 seeds/pot, 5 replicates for each treatments) in a water bath located in a growth chamber and grown while being watered with the solution twice a week in the following conditions for 60 days: 25 °C, 16/8-hour light/dark photoperiod, 350 µmol m-2 s-1 light intensity and 60% relative humidity. Water baths were used to keep the temperature of the media constant. The temperature of the media in which the plants grew was measured daily with a thermometer to verify whether the temperature was 25 °C. Afterward, half of these 60-day-old plants were kept growing at 25 °C and the other half were exposed to heat stress at 40 ºC for another 7 days (On-line Suppl. Fig. 1). Although H. thermophilum plants grow in soil at temperatures between 55-65 °C in their natural habitat, our preliminary experiment showed that in a liquid media the best growth performance was obtained at 40 °C. At higher temperatures liquid media is lost due to evaporation, and pH is unstable causing retardation of growth. In order to determine the presence of sulfur deficiency, attention was paid to the parameters of chlorosis in plants (pale green chlorosis), lack of green color in the veins or in many cases paler than the interveinal tissue, and earlier or more severe yellowing of young leaves than of mature leaves. At the end of the applications, measurements were made with the leaves of the plants.
Plant fresh weight
The fresh weights of the whole plants grown in the above-mentioned conditions were determined with precision scales at the end of the applications.
Relative water, proline and total soluble sugar contents
Relative water, proline and total soluble sugar contents were determined based on Saglam et al (2008). After measuring the fresh weight of leaves, plants were kept in deionized water at 4 °C for 24 h and the turgid weights were weighed. Afterward, the samples were kept in the oven set at 65 °C for 48 h, their dry weights were recorded, and their relative water content (RWC) was determined according to the formula:
RWC (%) = (fresh weight − dry weight / turgid weight − dry weight) × 100
For determination of proline content, 0.1 g of fresh plant samples were homogenized in 40% ethanol, incubated overnight in a micromixer at 300 rpm at +4 °C and then filtered. One mL of the filtrate was taken and mixed with 2 mL of reaction mixture containing 1 mL of 60% acetic acid, 20% ethanol, and 1% (w/v) ninhydrin. The samples were placed in tubes and kept in a water bath at 95 °C for 20 min, and the reaction was terminated on ice. The cooled samples were read in the spectrophotometer at 520 nm. The amount of proline was determined from a standard curve in the range of 20–100 µg.
To determine total soluble sugars, oven-dried leaves (0.1 g) were homogenized with 5 mL of 70% ethanol. The homogenate was boiled at 80 °C for 3 min and then cooled to room temperature and centrifuged at 10000 g for 5 min. For spectrophotometric determination, 100 μL of the supernatant was diluted by adding 900 μL of distilled water. One mL of 5% phenol was added to 1 mL of the sample and vortexed. After that, 5 mL of 96% sulfuric acid was added to the mix and then vortexed. The tubes containing the mixture were cooled to room temperature and their absorbance was measured at 490 nm by a spectrophotometer. The standard curve was made from 0, 20, 40, 60, 80, and 100 μg mL-1 glucose.
Hydrogen peroxide content and antioxidant enzyme activities
The content of hydrogen peroxide (H2O2) and antioxidant enzyme activities were determined according to Sağlam et al (2011).
To assess H2O2 content, 0.25 g of leaf samples was ground in 5 mL of 0.1% (w/v) trichloroacetic acid. The homogenate was centrifuged at 15000 g at 4 °C for 15 min. After the removal of 1 mL of the supernatant, 10 mM potassium phosphate buffer and 1 M potassium iodide were added, and the absorption of the mixture was measured at 390 nm. The hydrogen peroxide was determined from the standard curve of known concentration of hydrogen peroxide ranging from 0-100 nM.
For measurements of antioxidant enzymes activity, leaf samples (0.1 g) were powdered with liquid nitrogen and then extracted in 5 mL of extraction buffer (50 mM dipotassium hydrogen phosphate (K2HPO4), 1 mM ethylenediaminetetraacetic acid (EDTA) pH 7.0, 1% (w/v) polyvinylpyrrolidone (PVPP). The extracts were centrifuged at 20000 g at +4 °C for 20 min. The antioxidant enzyme activities were measured in the obtained supernatant, except for ascorbate peroxidase (APX) where 5 mM ascorbic acid was added to the extraction buffer.
Peroxidase (POD, EC 1.11.1.7) activity was assessed in 1 mL of the reaction mixture, which consisted of 100 mM potassium phosphate buffer (pH 7.0), 0.1 mM EDTA, 5 mM guaiacol, 15 mM H2O2, and 50 µL of enzyme extract, at a wavelength of 470 nm for a duration of 1 min. The POD activity was calculated by employing the extinction coefficient of 26.6 mM-1 cm-1 for tetraguaiacol, a colored product of enzymatic reaction.
Catalase (CAT, EC 1.11.1.6) activity was determined by measuring 1 mL of the reaction mixture containing 50 mM potassium phosphate buffer (pH 7.0), 30 mM H2O2, and 100 µL enzyme extract at 240 nm for 3 min. The CAT activity was calculated using the extinction coefficient of 39.4 mM-1cm-1 for H2O2.
To determine the activity of glutathione reductase (GR, EC 1.6.4.2), 50 µL of enzyme extract was added to a mixture consisting of 50 mM tris(hydroxymethyl)aminomethane hydrochloride (pH 7.8), 250 µL of 1 mM L-glutathione oxidized (GSSG), and 500 µL of 0.25 mM NADPH, which was prepared in 200 µL of 0.5 mM EDTA. The reduction of NADPH was measured by following the oxidation for a duration of 5 min at a wavelength of 340 nm. The enzyme activity was calculated using the extinction coefficient of NADPH, which is 6.22 mM-1 cm-1.
Ascorbate peroxidase (APX, EC 1.11.1.11) activity was determined based on the decrease in absorbance at 290 nm. The determination of enzyme activity involved the measurement of a 1 mL reaction mixture composed of 50 mM potassium phosphate buffer (with a pH of 7.0), 250 µM ascorbate (ASC), 5 mM H2O2, and 100 µL enzyme extract. The activity of APX was calculated by employing the extinction coefficient of 2.8 mM-1 cm-1 for ASC.
Protein determination
Determination of protein concentration was made spectrophotometrically (Thermo Scientific, Evolution 201 UV-VIS Spectrophotometer) according to Bradford (1976). By preparing bovine serum albumin standards (20-140 µg protein), the complex formed by proteins was measured at 595 nm with Coomassie Brilliant Blue G250 dye. Protein concentration was calculated in mg per liter and used to express enzyme activities.
RNA extraction and cDNA synthesis
Fresh leaf samples (0.1 g) were frozen with liquid nitrogen and thoroughly lysed with a tissue shredder. Afterwards, the protocol of the FavorPrep Plant Total RNA Purification Mini Kit (Favorgen Biotech Corp) was applied to obtain total RNA. The amount and purity of RNA in the samples were determined spectrophotometrically by Nanodrop (Thermo Scientific, NanoDrop 2000). Using a High Capacity Cdna Reverse Transcription Kit (Applied Biosystems) from isolated total RNA samples, cDNA was obtained at 2000 ng.
Quantitative PCR (qPCR)
Analyses were completed on the CFX Connect Real Time PCR System (BioRad) device using EvaGreen qPCR Supermix (Solis Biodyne) solution with cDNA to determine the gene expressions. The analysis was carried out by modifying the Solis Biodyne instructions and following the steps: The qPCR reaction program was set as follows: initial activation at 95 °C for 12 s; then denaturation at 95 °C for 15 s, annealing at 60 °C for 30 s, and extension at 72 °C for 30 s, repeated for 44 cycles; and a melting curve protocol (65-95 °C with fluorescence measured every 0.5 °C). Three biological replications were adopted for each sample. Gene-specific primers for qPCR analysis were created using the primer 3.0 online tool (http://bioinfo.ut.ee/primer3). The primers were used to examine the expression levels of actin 7 (reference gene) and HSFA4A, HSF4, and HSFA3 genes. Actin 7 was used to normalize the data obtained from the analysis and present it as the relative gene expression. The 2−∆∆Cq method was used to calculate gene expression (Bookout and Mangelsdorf, 2003). The primer sequences (5'-3') are as listed below:
ACT 7: Reference gene (ACT 7-F: TACGAGCAGGAGCTTGACAC, ACT 7-R: CCGATCATGGAAGGCTGGAA),
HSFA4A: Heat shock factor a4a (HSFA4A-F: TTCACCGACGCAAGCCAATA, HSFA4A-R: TTATGCCTCTCAAGCTCCGC),
HSF4: Heat shock factor 4 (HSF4-F: GCTTCGTTCGCCAGCTTAAC, HSF4-R: GCTTCGTTCGCCAGCTTAAC),
HSFA3: Heat shock factor a3 (HSFA3-F: CCTCCTAATAGTACGCCGCC, HSFA3-R: CAAGGGGAGAGGCCATTGTT).
Sulfur analysis
Plant samples were dried at 105 °C and ground using a mill (Sinbo SCM-2934). An elemental analyzer (LECO 628) burned 1.0 g of sample at a 300 mL min-1 flow rate at 1050 °C. The resulting SO2 was measured in the infrared cell of the elemental analyzer (Maj et al. 2020).
Statistical analysis
The statistical software SPSS version 23.0 was used to conduct two-way analysis of variance (ANOVA) on all the data. All the traits were examined in order to determine how high temperature, sulfur deficiency, and their interactions affect them. With the use of post hoc Tukey's multiple range test, the significance of differences between mean values (P < 0.05) was confirmed. The mean values for five replicates ± standard deviation (SD) were presented.
Results
When plants were given low amounts of sulfate and were exposed to high temperature, as opposed to complete nutrient delivery at 40 °C, the indications of sulfur deficiency, such as chlorosis, green color retention in the veins, and yellowing of young leaves were clearly visible (Fig. 1).
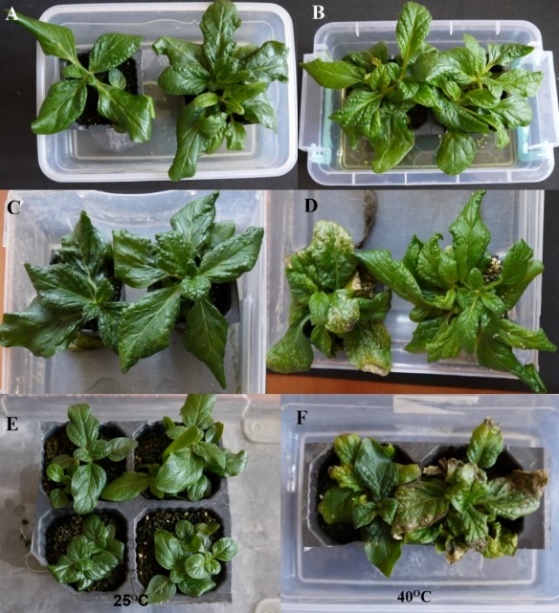
Fig. 1. Plants grown in full nutrition (FN) medium at (A) 25 ºC and (B) 40 ºC; 0.30 mM sulfate medium at (C) 25 ºC and (D) 40 ºC, and 0.15 mM sulfate medium at (E) 25 ºC and (F) 40 ºC. Plants were grown 60 days at 25 ºC in FN, 0.30 mM or 0.15 mM sulfate media and then subjected to a temperature of 40 ºC for 7 days.
Measurement of sulfur content confirmed that plants grown in both low-sulfate media experienced a decrease in sulfur content; the percentages of sulfur in plants growing at 25 °C in FN medium and in media containing 0.30 and 0.15 mM SO42- (later in the text 0.30 and 0.15 mM sulfate media) were 0.73%, 0.67%, and 0.63%, respectively. Compared to the plants at 25 °C, the S content was greater in plants at 40 °C; it was 0.77% and 0.79% in plants grown in the 0.30 and 0.15 mM sulfate media, while an even higher S level (0.82%) was measured in plants in the FN media.
The two-way ANOVA indicated highly significant (P < 0.01) effects of temperature, sulfur and the temperature × sulfur interaction on the plant fresh weight (Tab. 1).
Tab. 1. Two-way ANOVA (F values and statistical significance) considering the effect of treatment, variety, and interactions on the measured variables, as indicated. ns, **, *** indicate non-significant or significant at P < 0.05 and P < 0.01, respectively. Values in parentheses are degrees of freedom.
The fresh weight of the plants grown at 25 °C in 0.15 mM sulfate medium was lower than that of those grown in FN or 0.30 mM sulfate media. In addition, the plants in 0.15 mM sulfate medium were smaller than the plants in 0.30 mM sulfate and FN media. When high temperatures were applied, the fresh weights of plants grown in 0.30 and 0.15 mM sulfate media decreased compared with those grown in FN media (Fig. 2A). The high temperature treatment resulted in an increase in the fresh weight of plants in FN medium, while in 0.30 and 0.15 mM sulfate grown plants it was reduced by 39% and 20%, respectively, compared to respective values obtained at 25 °C.
The RWC was significantly affected by temperature, sulfur, and the temperature × sulfur interaction (P < 0.01) as demonstrated by the two-way ANOVA (Tab. 1). At both temperature, 25 and 40 °C, the RWC of the plants grown in 0.15 mM sulfate was lower than in plants grown in FN and 0.30 mM sulfate media (Fig. 2B). RWC of 0.15 mM sulfate-grown plants at 40 °C was reduced by 4.3% compared to the RWC of the plants at 25 °C. RWC values of FN and 0.30 mM-sulfate grown plants at 40 °C were not different from respective values at 25 °C.
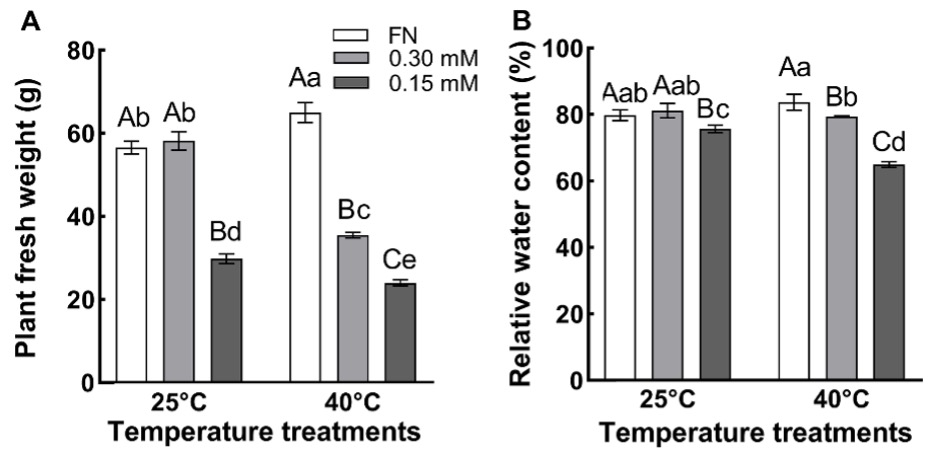
Fig. 2. The fresh weight (A) and the relative water content (B) of the plants grown at 25 ºC and 40 ºC in full nutrition (FN), 0.30 mM and 0.15 mM sulfate media. Data show the means ± the standard deviation of five independent samples. Different small letters indicate that means were statistically different (P < 0.05). Different capital letters indicate differences in different sulfate treatments at same temperature group (P < 0.05). Tukey’s multiple testing method was used to compare the means. Plants were grown 60 days at 25 ºC in FN, 0.30 mM or 0.15 mM sulfate media and then subjected to 40 ºC for 7 days.
Two-way ANOVA indicated highly significant (P < 0.01) effects of temperature, sulfur and the temperature × sulfur interaction on H2O2 content (Tab. 1). At 25 °C, the H2O2 level was found to be equal in plants grown in FN and 0.30 mM sulfate media, but significantly higher than in plants grown in 0.15 mM sulfate medium. The highest level of H2O2 was observed in plants grown in 0.15 mM sulfate medium at 40 °C (Fig. 3A). H2O2 level of plants grown in FN was reduced by 43% by heat treatment compared to the value of FN at 25 °C, while the H2O2 contents of the plants grown in 0.30 and 0.15 mM sulfate media at 40 °C were 39% and 105% higher than in those grown in 0.30 and 0.15 mM sulfate media at 25 °C.
The proline content was significantly (P < 0.01) affected by temperature, sulfur, and the temperature × sulfur interaction in two-way ANOVA (Tab. 1). The proline levels of plants grown in 0.15 and 0.30 mM sulfate media at 25 °C did not differ, but it was higher than in the plants grown in FN media. An increase in the proline content was evident in plants exposed to high temperature since the values in plants grown in FN, 0.30 mM, or 0.15 mM sulfate media increased by 10%, 26%, and 220%, respectively, compared to the respective values from the plants in FN, 0.30 and 0.15 mM sulfate media at 25 °C. Besides, the plants that were grown in 0.15 mM sulfate medium at 40 °C had a higher proline content than those grown in FN or 0.30 mM sulfate media (Fig. 3B).
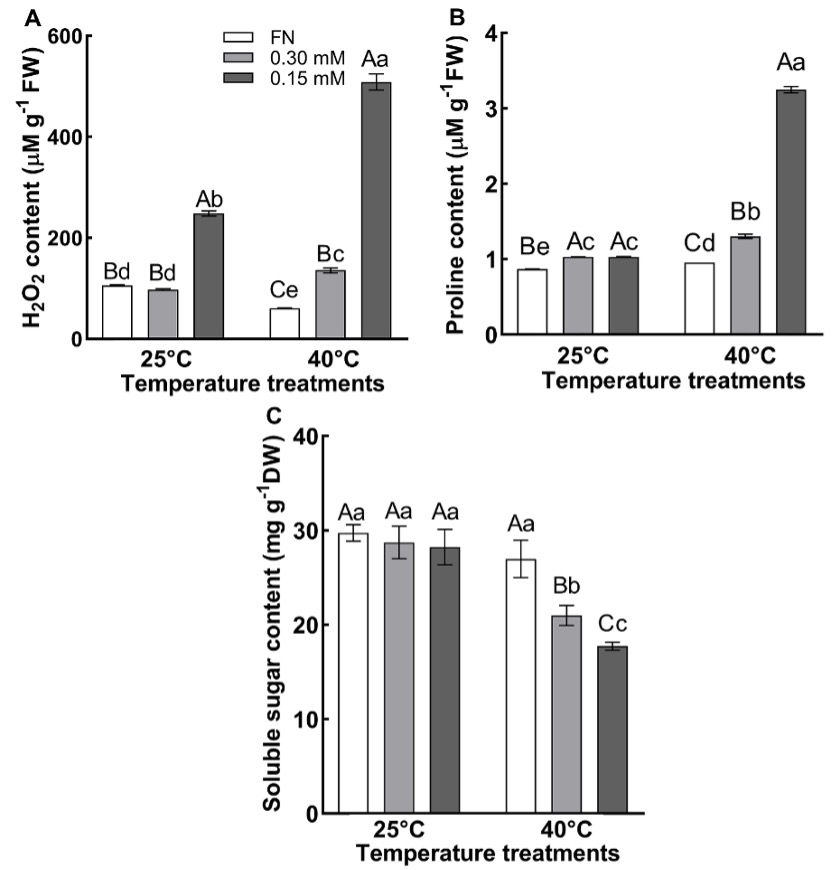
Fig. 3. The level of hydrogen peroxide (H2O2) (A), proline (B), and total soluble sugar (C) of the plants grown at 25 ºC and 40 ºC in full nutrition (FN), 0.30 mM and 0.15 mM sulfate media Data show the means ± the standard deviation of five independent samples. Different small letters indicate that means were statistically different (P < 0.05). Different capital letters indicate differences in different sulfate treatments at same temperature group (P < 0.05). Tukey’s multiple testing method was used to compare the means. Plants were grown 60 days at 25 ºC in FN, 0.30 mM and 0.15 mM sulfate media then subjected to a temperature of 40 ºC for 7 days.
In the two-way ANOVA (Tab. 1), temperature, sulfur, and the temperature × sulfur interaction all affected the soluble sugar content significantly (P < 0.01). The total soluble sugar content of plants grown in FN, 0.30 and 0.15 mM sulfate media did not differ at 25 °C. At high temperature (40 °C), the sugar content of plants grown in FN medium was similar to that in plants at 25 °C, while in plants grown in 0.30 and 0.15 mM sulfate media it was reduced by 27% and 36% compared to the plants grown in 0.30 and 0.15 mM sulfate media. Also, at 40 °C sugar content in plants grown in 0.30 and 0.15 mM sulfate media was lower than in FN medium grown plants at 40 °C (Fig. 3C).
Two-way ANOVA showed significant effects of sulfur deficiency and the temperature × sulfur interaction on APX activity (P < 0.01) while no significant effect was observed for high temperature on the enzyme activity (Tab. 1). The APX activity of the plants grown in FN medium was higher than that of those grown in 0.30 and 0.15 mM sulfate media at 25 °C. (Fig. 4A). The APX activities in FN medium-grown plants at 25 °C and 40 °C were not different. However, APX activity was induced by low sulfur and high temperature treatments. The APX activity of plants grown in 0.30 and 0.15 mM sulfate media at 40 °C was increased by 25 and 83%, respectively, compared to values of the plants grown in 0.30 and 0.15 mM sulfate media at 25 °C. In addition, the activity in the plants grown in 0.15 mM sulfate medium was lower than in the plants grown in 0.30 mM sulfate medium at 40 °C (Fig. 4A).
Temperature, sulfur, and the temperature × sulfur interaction affected the GR activity significantly (P < 0.01) according to two-way ANOVA (Tab. 1). The GR activity of plants grown in 0.30 mM sulfate at 25 °C was found to be significantly higher than that of plants grown in FN and 0.15 mM sulfate media at 25 °C (Fig. 4B). GR activity decreased by 56% in plants grown in 0.30 mM sulfate medium at 40 °C compared to value of the plants grown in 0.30 mM sulfate medium at 25 °C. At 40 °C, GR activity was found to be higher in plants grown in FN medium compared to the plants grown in 0.30 and 0.15 mM sulfate media (Fig. 4B).
Two-way ANOVA showed highly significant effects of sulfur deficiency and the temperature × sulfur interaction on CAT activity (P < 0.01) and a significant effect of temperature (P < 0.05). While CAT activity was similar in the plants grown in FN and 0.15 mM sulfate media at 25 °C, it was higher in the plants grown in 0.30 mM sulfate medium. Under high-temperature conditions, CAT activity significantly increased in plants grown in 0.30 mM sulfate medium compared to plants grown in 0.30 mM sulfate medium at 25 °C. In addition, the CAT activity of the plants in 0.30 mM sulfate medium at 40 °C was higher than that of the plants in FN and 0.15 mM sulfate medium (Fig. 4C). At 40 °C, the CAT activity in 0.30 mM-sulfate grown plants increased by 16% while in 0.15 mM-sulfate grown plants it decreased by 46% compared to the plants grown in the same media (0.30 mM and 0.15 mM) at 25 °C, respectively.
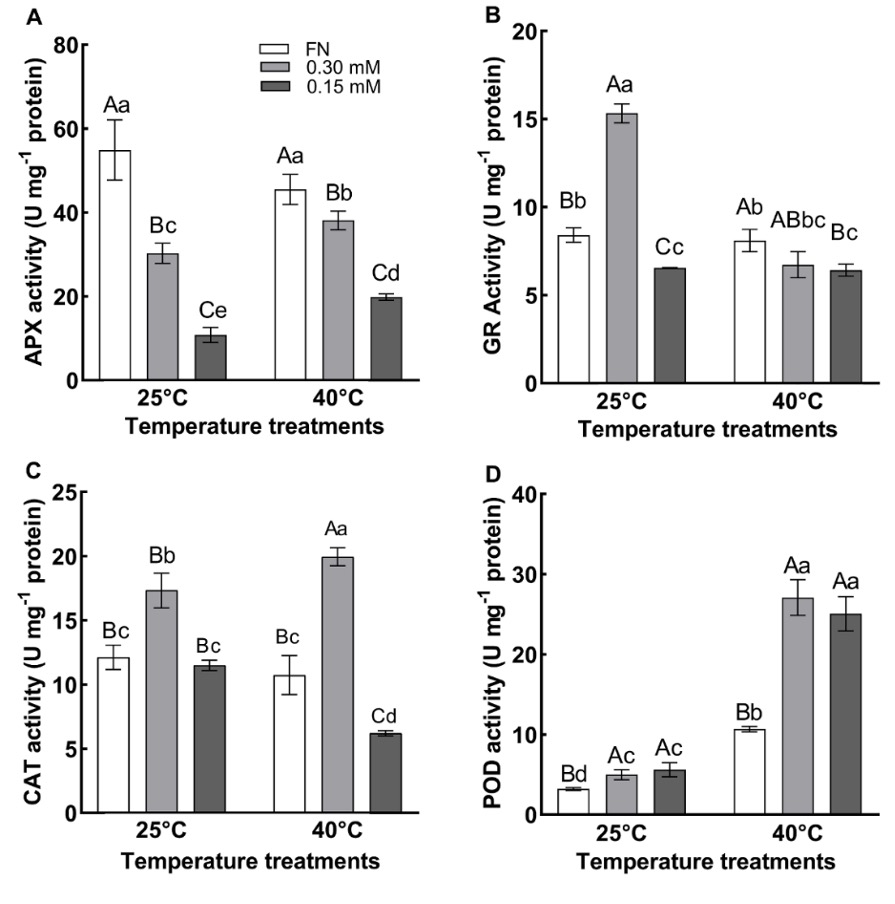
Fig. 4. Activities of ascorbate peroxidase (APX) (A), glutathione reductase (GR) (B), catalase (CAT) (C) and peroxidase (POD) of the plants grown at 25 ºC and 40 ºC in full nutrition (FN), 0.30 mM and 0.15 mM sulfate media. Data show the means ± the standard deviation of five independent samples. Different small letters indicate that means were statistically different (P < 0.05). Different capital letters indicate differences in different sulfate treatments at same temperature group (P < 0.05). Tukey’s multiple testing method was used to compare the means. Plants were grown 60 days at 25 ºC in FN, 0.30 mM and 0.15 mM sulfate media then subjected to a temperature of 40 ºC for 7 days.
The POD activity was significantly (P < 0.01) affected by temperature, sulfur, and the temperature × sulfur interaction in two-way ANOVA (Tab. 1). At 25 °C, the POD activity did not differ between plants grown in 0.30 mM and 0.15 mM sulfate media. However, they showed higher activities than plants grown in FN medium at 25 °C (Fig. 4D). Plants with high-temperature conditions showed a significant increase in POD activity compared to plants with 25 °C conditions. POD activity in the plants grown in FN, 0.30 and 0.15 mM sulfate media at 40 °C increased by 234%, 442%, and 348% respectively, compared to the corresponding values obtained from the plants grown in FN, 0.30 and 0.15 mM sulfate media at 25 °C. At 40 °C, there was no significant difference in POD activity between plants grown in 0.30 and 0.15 mM sulfate media. However, these groups had higher POD activity than plants grown in FN medium (Fig. 4D).
HSF4 expression was affected significantly (P < 0.01) by temperature, sulfur, and the temperature × sulfur interaction according to the two-way ANOVA (Tab. 1). The expression level of the HSF4 gene in the plants grown in 0.30 mM sulfate medium was lower at 25 °C than plants grown in FN medium and 0.15 mM-sulfate grown plants. Moreover, it was the highest in 0.15 mM-sulfate grown plants. In addition, the HSF4 gene expression level increased with increasing temperature in all sulfate concentrations (Fig. 5A); the plants grown in FN, 0.30 and 0.15 mM sulfate media experienced a 9.9, 194, and 20-fold increase in HSF4 gene expression, respectively compared to corresponding values of the plants grown in FN, 0.30 mM and 0.15 mM sulfate media at 25 °C. At 40 °C, the expression level was highest in plants grown in 0.30 mM sulfate medium and the lowest in FN medium-grown plants (Fig. 5A).
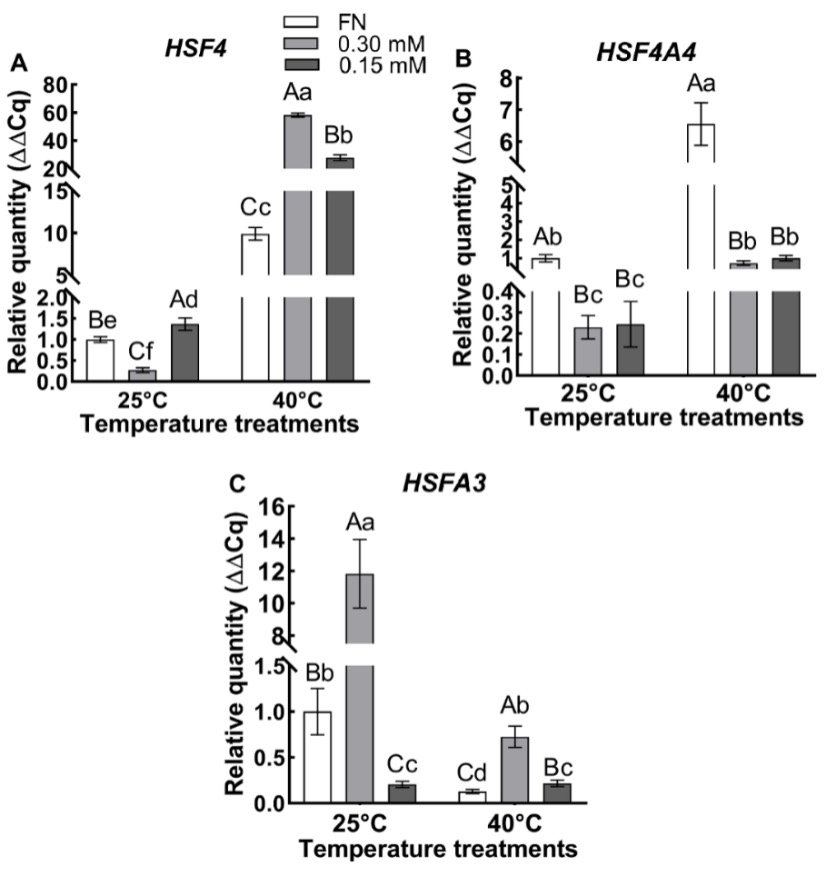
Fig. 5. The expression level of the HSF4 (A), HSF4A4 (B) and HSFA3 (C) genes of the plants grown at 25 ºC and 40 ºC in full nutrition (FN), 0.30 mM and 0.15 mM sulfate media. Data show the means ± the standard deviation of five independent samples. Different small letters indicate that means were statistically different (P < 0.05). Different capital letters indicate differences in different sulfate treatments in the same temperature group (P < 0.05). Tukey’s multiple testing method was used to compare the means. Plants were grown 60 days at 25 ºC in FN, 0.30 mM and 0.15 mM sulfate media then subjected to a temperature of 40 ºC for 7 days.
Two-way ANOVA (Tab. 1) demonstrated a very significant (P < 0.01) effect on HSFA4A expression due to temperature, sulfur, and the temperature × sulfur interaction. At 25 °C, the HSFA4A gene expression level of FN-medium grown plants was higher than that of plants grown in 0.30 mM and 0.15 mM sulfate media. Under all sulfate conditions, gene expression increased when plants were exposed to a temperature at 40 °C i.e. plants grown in FN, 0.30 mM, and 0.15 mM sulfate media experienced a 6.6, 3.2, and 7.3-fold increase in HSFA4A gene expression, respectively, compared to respective values obtained at 25 °C. HSFA4A gene expression levels were similar in plants grown in 0.30 and 0.15 mM sulfate media at 40 °C (Fig. 5B). Moreover, sulfur-deficient plants had lower expression levels than FN-medium grown plants.
The two-way ANOVA (Tab. 1) demonstrated that HSFA3 expression was significantly (P < 0.01) influenced by temperature, sulfur, and the interaction between temperature and sulfur. When the temperature increased, the expression level of HSFA3 in plants grown in FN and 0.30 mM-sulfate media decreased by 7.7- and 16.2-fold, respectively, compared to the respective values of the plants grown in FN and 0.30 mM-sulfate media at 25 °C. Furthermore, the gene expression level in 0.15 mM-sulfate medium grown plants was similar and the lowest at both 25 °C and 40 °C (Fig. 6C).
Discussion
Plant growth parameters such as fresh weight are affected negatively by high temperature (Ihsan et al. 2019). Sezgin Muslu and Kadıoğlu (2021) found a decrease in H. thermophilum's shoot fresh weight at soil temperatures exceeding 80 °C. In our study, H. thermophilum plants were exposed to two distinct sulfate concentrations to evaluate the impact of sulfur deficiency on their response to high temperatures in vermiculite media with Hoagland nutrient solution. At 40 °C, the plants treated with low sulfate concentrations experienced a greater decrease in fresh weight than the plants at 25 °C. Sulfur deficiency has been found to have an impact on plant biomass, overall morphology, yield, and nutritional value. For instance, in Eruca sativa, sulfur deficiency resulted in a reduction in biomass production and chlorophyll synthesis (Narayan et al. 2023). Sulfur deficiency may also cause a decrease in the synthesis of sulfur-containing protein and amino acids (Ali et al. 2019), which may affect the growth of H. thermophilum in our study especially during stress caused by higher temperature.
The water content was shown to decrease in plants at high temperatures (Gupta et al. 2018). The RWC at various sulfate concentrations and temperatures was determined in Heliotropium plants. FN and 0.30 mM sulfate did not affect RWC, but 0.15 mM sulfate at 25 °C decreased it. Sulfur deficit has been found to impede stomatal closure in plants (Ren et al. 2022). Besides stomatal closure being hindered by the increase in sulfur deficiency it is also possible that the water content could have decreased due to tissue damage in the leaves. As in our findings, tissue damage and consequent water loss occurred in pyrethrum flowers subjected to drought and high temperature of 40 °C (Carins-Murphy et al. 2023). Since in our study there was no change in water content when there was enough sulfur, we may conclude that the decrease in water content under conditions of 0.15 mM sulfate concentration may be attributable to a shortage of sulfur rather than high temperature.
In our study, we found morphological alterations such as chlorosis, immature leaves being paler than adult leaves, and an inability to keep the green color in leaf veins in plants treated with low sulfate concentrations at high temperatures. These changes revealed that the leaves' stress level increased when the temperature was applied together with low sulfate concentration. Tian et al (2009) observed that Agrostis scarba was not damaged by high temperature when applied for 12 days at 35 °C in the absence of sulfur deficiency. Similarly, Sezgin Muslu and Kadıoğlu (2021) did not report morphological damage in H. thermophilum caused by high soil temperature at temperatures of 40, 60, and 80 °C compared to the control (20 °C) when adequate sulfate concentration was present. According to Chung et al. (2022), structural problems in the iron-sulfur protein, which is involved in the formation of chlorophyll, could be involved in the sulfur deficiency-induced chlorosis in our study. When plants are subjected to high temperatures, they not only lose chlorophyll but also experience an increase in H2O2 content (Sezgin Muslu and Kadıoğlu, 2021). Additionally, it was noted that mulberry and maize undergo an increase in H2O2 due to sulfur deficiency (Tewari et al. 2010). In our investigation, we discovered an increase in H2O2 concentration in response to increased sulfur deficiency and high temperature. The maximum concentration of H2O2 was found in plants grown at 0.15 mM sulfate and exposed to 40 °C. This result showed that the balance between H2O2 production and breakdown was disturbed. Moreover, the application of 0.15 mM sulfate on plants resulted in low activity of antioxidant enzymes APX, CAT, and GR compared to FN in which enough sulfate was supplied. The insufficiency of sulfur induces the oxidation of biomolecules as a result of the excessive buildup of ROS and diminished production of compounds containing sulfur, such as GSH, thioredoxin, and glutaredoxin, which can efficiently form a line of defense against reactive oxygen and nitrogen species (Hill et al. 2022). In line with that, we observed higher GR and CAT activities in the plants subjected to 0.30 mM sulfate at 25 °C. The GR activity, which converts GSSH, may be increased to replace the decreased GSH due to sulfur deficiency and CAT activity may be triggered to remove H2O2. Therefore, the plants tried to keep levels of the metabolites related to sulfur metabolism, such as cysteine and methionine, at a constant level under stress conditions. In addition, at 40 °C in plants grown at 0.30 mM sulfate, APX and CAT activities increased compared to plants grown at 25 °C. However, there was a visible downward trend for GR when high temperature was applied. Bashir et al. (2015) reported that Brassica juncea plants that were subjected to low sulfate (30 μM SO42−) become sensitive to cadmium toxicity. Besides, Wang et al. (2021) discovered that when Brassica rapa plants were grown in low sulfur environments, there were notable reductions in GSH content and GR activity when compared to the control group. In summary, sulfur shortage negatively affected the antioxidant system which is necessary for the thermotolerance of H. thermophilum.
In addition to antioxidant enzymes, plants also build up substances known as osmolytes such as proline and soluble sugars to lessen the impacts of stresses. Proline has been shown to have functions beyond osmotic regulation, including protecting membrane integrity, stabilizing proteins, and regulating cytosol pH (Mehta and Vyas, 2023). The highest amount of proline was found in plants that were treated with 0.15 mM sulfate at 40 °C temperature during our study. The accumulation of proline was increased in order to reduce temperature sensitivity due to the decrease in sulfate concentration. Various species, such as Vigna unguiculata, Cicer arietinum, Solanum lycopersicum, and Gossypium hirsutum, have been documented to exhibit proline accumulation in response to elevated temperatures (Du et al. 2011). However, the severity of high temperature was a significant factor in the extent of proline accumulation. An elevated proline level caused by a sulfur shortage and high temperature in our study was a sign of mounting stress. In addition, a rise in proline levels may support the preservation of the cytoplasmic osmotic potential of cells, a critical process for plant survival in stressful environments.
Sugar builds up in plant tissues in a manner similar to that of proline to protect biomolecules and membranes by regulating the intrinsic osmotic potential (Mehta and Vyas, 2023). Sugar accumulation under stress has been documented in numerous species and is believed to exert a significant influence on thermal tolerance (Zhao et al. 2022). Zheng et al. (2022) found that the soluble sugar contents of tomato plants subject to high temperature (41 °C) diminished due to the inhibition of sucrose synthase. In our study, we observed a decrease in the soluble sugar content in sulfur deficiency media under heat stress which could be a result of either inhibition of sugar biosynthesis enzymes or a shift in metabolism to the synthesis of antioxidant compounds or proline instead of sugar synthesis. Yu et al. (2022) reported that sulfur deprivation leads to a decrease in glucose content in Arabidopsis thaliana. Furthermore, Wawrzyńska et al. (2022) discovered that when a sulfur deficiency occurred, the production of anthocyanin was triggered while the photosynthesis rate and sugar production were slowed down.
HSFs are the primary factor responsible for activating HSP genes in response to heat stress. The activation of HSFA2, a transcriptional activator of HSP expression, and HSP17-CII has been documented in the anther of the tomato plant during its growth in elevated temperatures (Giorno et al. 2010). The thermotolerance of transgenic soybeans may be amplified by the over-expression of GmHSFA1 in soybeans, potentially by activating downstream genes like GmHsp70, GmHsp22, and other GmHsps in response to heat stress (Zhu et al. 2006). In Arabidopsis, HsfB1 (also known as HSF4 or TBF1) suppresses the overall heat shock response in the absence of high heat but promotes the development of acquired thermotolerance during heat stress (Pick et al. 2012). In the current study, HSF4 and HSFA4A genes were up-regulated at 40 °C in all sulfate treatments (FN, 0.30 mM sulfate and 0.15 mM sulfate) compared to the values at 25 °C. At 0.30 mM sulfate, the rate of HSF4 gene expression was the highest after high-temperature treatment which probably changed the expression levels of various genes to resist heat and sulfur deficiency stress. Lin et al. (2018) reported that HSFA2 and HSFA4a control the transcription of HSPs to establish the heat shock response in A. thaliana seedlings subjected to 39 °C. Induction of HSFA4 genes in H. thermophilum under heat stress was the greatest in FN plants but much lower under sulfur deficiency which may have negatively affected plant resistance to high temperatures. The expression level of the HSFA3, which is a pivotal heat shock factor that interacts with HSFA2 to effectively enhance transcriptional memory in response to heat stress (Friedrich et al. 2021), was lower in the FN plants and plants at 0.15 mM sulfate compared to those supplied with 0.30 mM sulfate at 25 °C. The induction of HSFA3 in the plants subjected to 0.30 mM sulfate at 25 °C may be a result of sulfur deficiency while application of 0.15 mM sulfate may have disrupted the functioning of systems that control HSFA3 expression. However, the expression of HSFA3 genes in plants at 40 °C was lower than that in plants at 25 °C at all sulphate treatments which should be further investigated.
In summary, while plants growing in a full nutrition medium were tolerant to increasing temperatures, they became sensitive as the sulfate content decreased. As the dose level of sulfur deficiency increased, chlorophyll loss, injuries, and increased oxidative stress damage were observed in the leaves. The antioxidant system was negatively affected by sulfur deficiency. While plants preferred proline for osmoregulation, sugar production decreased. In addition, plants attempted to resist high-temperature damage caused by sulfur deficiency through the activation of the HSF4 and HSF4A4 genes, as well as the downregulation of HSFA3. However, more detailed studies on this subject are necessary.