Introduction
Salinity is one of the most important factors limiting the yield of crops, especially in arid, semi-arid, and coastal areas (Mostofa et al. 2015). This stress negatively affects plant physiology by imposing several major limitations leading to reduced crop growth and productivity (Ali et al. 2021, Zhang et al. 2021, Khan et al. 2023). Salinity causes osmotic and ionic stress, and production of reactive oxygen species (ROS) in plant cells (Ahammed et al. 2018, Khan et al. 2023, Colin et al. 2023). High production of ROS induces oxidative stress, which can destroy membrane components and biomolecules and, at higher levels, cause cell, tissue, and plant death (Kaur et al. 2022, Khan et al. 2023, Peng et al. 2023). Salinity tolerance is a complex process that involves various molecular, physiological, and biochemical mechanisms (Zhang and Shi 2013, Islam et al. 2023).
The use of osmoprotectants can augment stress tolerance mechanisms in plants (Chen and Jiang 2010, Islam et al. 2023). These compounds, which are known as compatible solutes, are highly soluble compounds with low molecular weight that directly or indirectly protect plants against stresses through various mechanisms such as regulating cellular osmosis, preventing membrane damage, stabilizing proteins and enzymes, and eliminating ROS (Singh et al. 2015, He et al. 2018). Application of exogenous osmoprotectants can be considered an alternative approach to improve plant productivity under saline conditions (Nakayama et al. 2005). The high concentration of compatible solutes can balance the salts entering the cell and at the same time deal with the high concentration of salts inside the cell (Türkan and Demiral 2009).
Sugars are one of the most important compatible solutes that, in addition to the role of signal molecules, can act as a source of metabolic energy and play a role in regulating metabolism in plants (Islam and Mohammad 2021). Trehalose (Tre) is a non-reducing disaccharide consisting of two glucose molecules with a chemically non-reactive nature that makes it compatible with cellular metabolism even at high concentrations, and plays an important role as an osmoprotectant in stress tolerance of some plants (Ali and Ashraf 2011, Lunn et al. 2014). It is naturally distributed in many organisms, from bacteria and fungi to plants and invertebrates (Nounjan et al. 2012, Kosar et al. 2019), where it is used for carbon storage and transport as well as being an osmoprotectant (Lunn et al. 2014). Among plants, Tre synthesis has been reported in some resurrection plants and other plants such as Arabidopsis (Muller et al. 1999, Lunn et al. 2014). It has been shown that the expression of plant genes involved in Tre metabolism undergoes remarkable changes in response to various abiotic stresses (Nakashima et al. 2009). Tre and trehalose-6-phosphate, a phosphorylated intermediate in Tre biosynthesis, act as signal molecules in carbohydrate metabolism (Wingler et al. 2000, Lunn et al. 2014). Tre induces sucrose synthase activity in soybean (Muller et al. 1998) and trehalose-6-phosphate acts as a specific signal of sucrose status in Arabidopsis, and may be seen as part of a homeostatic mechanism to control the level of sucrose, as well as part of regulatory networks in sucrose export in source organs and in growing sink organs (Lunn et al 2014). Tre has also a key role in the control of metabolism during plant growth and development, and reduces the effects of stress through mechanisms such as membrane protection, stabilization of proteins and enzymes, detoxification and removal of ROS, molecular signaling, and increasing the accumulation of osmolytes (Luo et al. 2010, Abdallah et al. 2016, Islam and Mohammad 2021). Most studies on the role of osmoprotectants in enhancing stress tolerance have focused on proline and glycine betaine, but there is relatively little knowledge about the effects of Tre on higher plants under stress conditions. Some studies have shown that the exogenous application of Tre on plants has induced tolerance to salinity stress (Nounjan et al. 2012, Abdallah et al. 2016, Rohman et al. 2019). In contrast, it has been found that Tre can have an inhibitory effect on the growth of some plants (Schluepmann et al. 2004, O'Hara et al. 2013) and algae under salinity (Panjekobi and Einali 2021). However, in most plants, endogenous Tre cannot adequately ameliorate the harmful effects of stress (Mostofa et al. 2015).
Basil ( Ocimum basilicum L.) is a medicinal and aromatic plant that is used in the pharmaceutical and food industries due to its abundant essential oils, high content of phytomedicines and natural antioxidant molecules, and is usually produced for economic purposes (Kwee and Niemeyer 2011, Caliskan et al. 2017). It is commonly used in traditional medicine and herbal therapy (Bahcesular et al. 2020, Farouk and Omar 2020). To date, no study has been conducted on the role of Tre in the physiological and biochemical responses of basil, an important agricultural and medicinal plant, to salinity stress. Accordingly, basil was taken as a model system to determine the effect of exogenous Tre on growth, accumulation of osmolytes, and the activity of some enzymes of the antioxidant system under salt stress.
Plant material and experimental design
Basil seeds ( Ocimum basilicum L.) obtained from the Botanic Garden at the University of Sistan and Baluchestan were sown in a greenhouse with a temperature of 29 ± 1 °C in trays containing moist cocopeat. After germination, seedlings with two or three leaves that had grown uniformly were selected and each seedling was transferred to a 14 x12 cm plastic pot containing 1 kg of cocopeat and placed at the same temperature. Seedlings were irrigated with 1/2 Hoagland's standard nutrient solution at three-day intervals until reaching the 6-8 leaf stage. Three days before salt treatment, the seedlings were divided into two parts. By foliar spraying on the seedlings, one part was treated with a concentration of 5 mM Tre and the other part received only distilled water. Salinity treatment was applied by adding sodium chloride (NaCl) in concentrations of 0, 25, 50, 100, and 150 mM to the nutrient solutions of each plant group. Tre-untreated plants, which received only 1/2 Hoagland's solution without NaCl, were considered as the control for salinity experiments. Seedlings were treated twice a week with salinity and every week with Tre for four weeks. After this period, seedlings were harvested and studied to investigate the morphological and biochemical responses to salt stress and Tre treatment. Each morphological or biochemical experiment was performed individually with three biological replicates.
Morphological traits
The length of root and shoot along with the length, width, and number of leaves of basil seedlings were measured. To determine the amount of biomass, first the fresh weight (FW) of each shoot and root was measured, and after exposure to 70 °C for 72 h, the dry weight (DW) was determined. Shoot water content (SWC) of basil seedlings was obtained using the following formula:
SWC = ((shoot FW− shoot DW)/shoot FW) × 100
Determination of photosynthetic pigments content
Photosynthetic pigments including chlorophyll (Chl) and total carotenoids (Car) were extracted from 1 g of fresh leaf tissue with 80% acetone. The resulting mixture was filtered through filter paper (Whatman No. 1) and the residue was saved to measure soluble sugars and total protein. The absorbance of the filtrate was recorded at 663, 645, and 652 nm, and the amounts of Chls a, b, and total were measured using the following equations (Arnon 1949):
Chl a (mg mL-1) = 0.0127 × A663 - 0.00269 × A645
Chl b (mg mL-1) = 0.0229 × A645 - 0.00468 × A663
Total Chl (mg mL-1) = A652/34.5
Total Car content was measured at 470 nm using the following equation (Lichtenthaler and Buschmann, 2001).
Total Car (μg mL-1) = (1000 × A470-1.82 × Chl a - 85.02 × Chl b)/198
The content of pigments was expressed as mg per g FW.
Determination of soluble sugars and starch content
Soluble sugars, including reducing and non-reducing sugars, were extracted from 40 mg of acetone powder (resulting from the extraction of photosynthetic pigments) using 80% ethanol (Einali and Valizadeh 2017). Ethanol extracts were used to determine the content of soluble sugars. Reducing sugars (RS) were determined by the method of Miller (1959) and non-reducing sugars (NRS) were measured by Handel's (1968) method. The residues obtained from the extraction of soluble sugars were used to extract and measure the amount of starch (McCready et al. 1950). The content of soluble sugars and starch was expressed as mg per g FW.
Determination of total proteins, total amino acid, and proline content
Total proteins refer to proteins extracted with a sample buffer containing 60 mM Tris–HCl buffer (pH 6.8), 10% (v/v) glycerol, and 2% (w/v) sodium dodecyl sulfate (Stone and Gifford (1997). Extraction of total proteins from 20 mg of acetone powder was done with 0.5 mL of sample buffer at 90 °C for 1 h, followed by centrifugation at 10 000 g for 15 minutes (Alisofi et al. 2020). The amount of total proteins was measured at a wavelength of 750 nm by the method of Markwell et al. (1981) and expressed as mg per g FW.
Free amino acids and proline were extracted from 0.2 g of fresh leaf tissue using 80% ethanol at 70 °C for 10 minutes, followed by centrifugation at 2 000 g for 10 minutes (Einali and Valizadeh 2017). The extraction process was repeated four more times. The concentrated ethanol extract was decolored by chloroform (1:5, v/v). The ninhydrin (1% (w/v) ninhydrin and 0.06% (w/v) KCN in acetone) method using a glycine calibration curve at 570 nm was used to determine total free amino acid (Yemm and Cocking 1955). Proline content was measured at 520 nm by another ninhydrin (1% (w/v) ninhydrin in 60% (v/v) acetic acid) method using proline as a standard (Bates et al. 1973). The content of free amino acids and proline was expressed as µmol per g FW.
Soluble protein extraction and enzyme assays
Crude enzyme extract was prepared from 0.2 g of fresh leaf tissue with 3 mL of enzyme extraction buffer containing 100 mM cold potassium phosphate buffer (pH 7.0), 10% glycerol, 1 mM EDTA, 10 mM KCl, 1 mM MgSO4, 1 mM phenylmethylsulphonyl fluoride (PMSF), 50 mM 2-mercaptoethanol, 0.1% (v/v) Triton X-100, and 1% (w/v) polyvinylpolypyrrolidone (PVPP)) as described elsewhere (Alisofi et al. 2020). Extraction was done in a cold mortar with pestle. The extraction buffer for ascorbate peroxidase (APX) determination contained 5 mM ascorbic acid as well. The homogenate was filtered through four layers of cheesecloth and incubated at 4 °C for 1 h. The amount of 10 mg of charcoal was added to the filter to remove the extracted pigments, and then it was centrifuged at 12 000 g for 10 minutes at 4 °C. The supernatant, containing soluble protein fraction, was used for enzyme assays. Soluble proteins refer to proteins extracted in the absence of sodium dodecyl sulfate. Soluble protein content was measured by Bradford's (1976) method using the albumin standard curve and expressed as mg per g FW.
APX reaction mixture (1 ml) consisted of 50 mM potassium phosphate buffer (pH 7.0), 1 mM H2O2, 0.5 mM ascorbic acid, and 50 µL enzyme extract. The oxidation of ascorbate to dehydroascorbate was monitored at 290 nm and the activity of the enzyme was calculated using the extinction coefficient 2.8 mM-1 cm-1 and expressed as µmol of oxidized ascorbate per min per g FW (Chen and Asada 1992).
The activity of pyrogallol peroxidase (PPX) was measured in a reaction mixture (1 mL) containing 50 mM potassium phosphate buffer (pH 7.0), 40 mM pyrogallol, 1 mM H2O2, and 50 µL enzyme extract. The conversion rate of pyrogallol to purpurogallin was monitored at 430 nm and the enzyme activities were calculated using the extinction coefficient 2.47 mM-1 cm-1 and expressed as µmol of purpurogallin produced per min per g FW (Nakano and Asada 1981). Polyphenol oxidase (PPO) activity was measured in the same way as PPX at 430 nm using the extinction coefficient 2.47 mM-1 cm-1, except that the reaction mixture was without H2O2 (Nakano and Asada 1981).
Statistical analysis
All results obtained from plant growth and biochemical studies were expressed as mean and standard deviation (SD) of three independent measurements. The statistically significant difference between treatments was determined in the form of factorial design using two-way ANOVA and Duncan's test at the level of 5% (P < 0.05).
Effect of salinity and Tre on plant growth
Salt treatment at low concentration (25 mM) caused a clear 15% increase in shoot length compared to the control, but higher salt concentrations gradually decreased these values (Tab. 1).
Tab. 1. Longitudinal growth and leaf characteristics of trehalose-treated (+Tre) or untreated (-Tre) basil seedlings in response to different salinity concentrations. Values are the mean ± standard deviation of three separate measurements. Different letters in each column indicate significant differences between the various treatments at P < 0.05 according to the Duncan test. LL - leaf length; LW - leaf weight.
However, salinity did not affect root length and leaf characteristics including length (LL), width (LW), LL/LW ratio, LL × LW production, and number of leaves. Tre treatment alone or under salt stress had a significant negative effect on the length of the shoot and some leaf characteristics compared to untreated controls, but it did not change the root length and the number of leaves (Tab. 1).
Although SWC remained unchanged, the effect of salinity on fresh and dry weight of shoots and fresh weight of roots was negative and caused a decrease proportional to salt concentration, while the dry weight of roots increased significantly in response to different salt treatments compared to the control (Tab. 2).
Tab. 2. Shoot and root biomass accumulation and water content of trehalose-treated (+Tre) or untreated (-Tre) basil seedlings in response to different salinity concentrations. Values are the mean ± standard deviation of three separate measurements. Different letters in each column indicate significant differences between the various treatments at P < 0.05 according to the Duncan test. FW - fresh weight; DW - dry weight; SWC - shoot water content.
Tre application in most salt treatments caused a decrease in fresh and dry weight of shoot and root, so that the decrease in dry weight was more intense. In contrast, Tre treatment caused a 23% increase in SWC in seedlings exposed to 150 mM NaCl compared to the untreated control (Tab. 2).
Effect of salinity and Tre on photosynthetic pigments
The amount of Chl and Car in response to salinity of 50 mM and above decreased significantly compared to the control (Fig. 1).
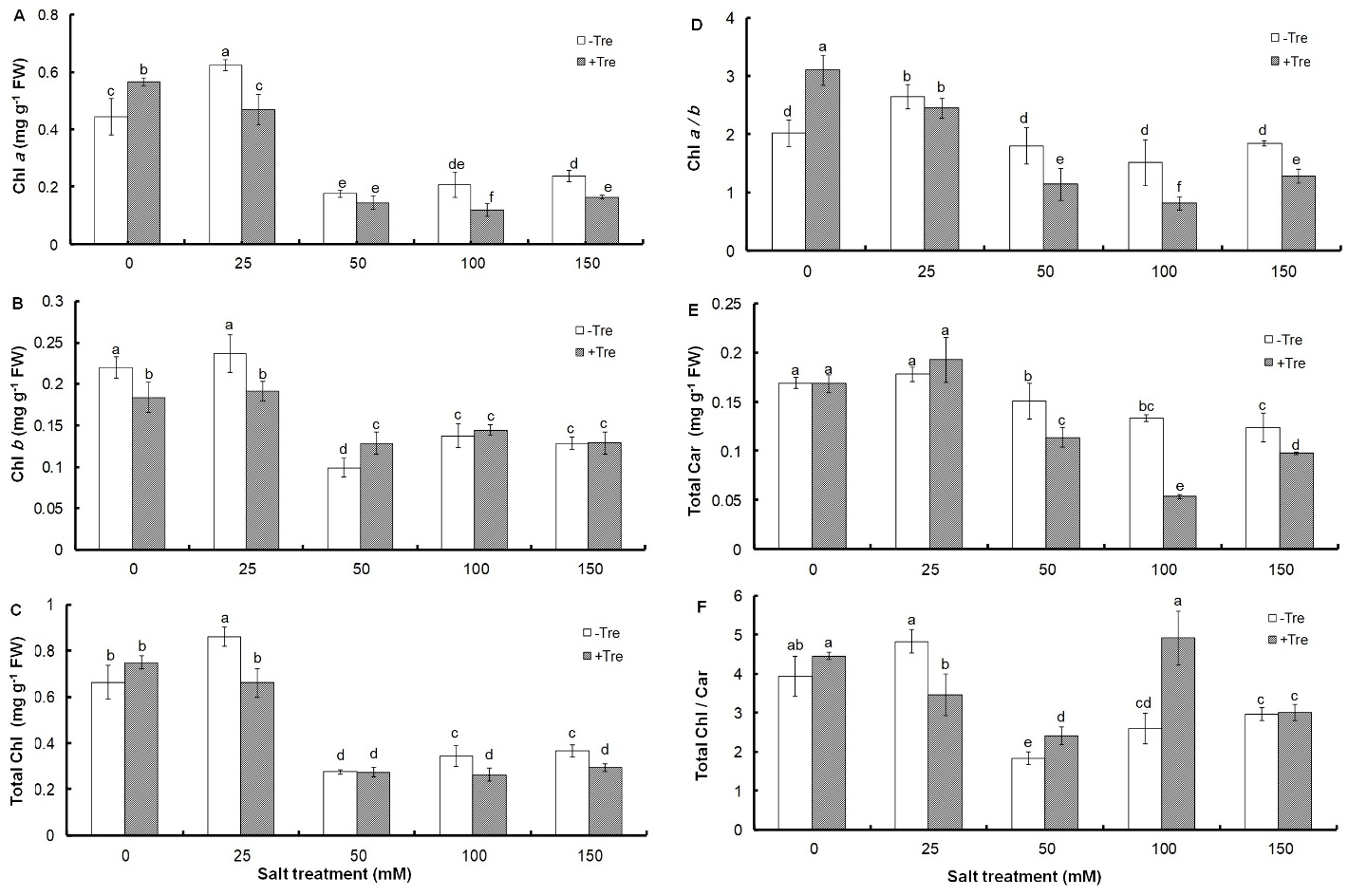
Fig. 1. Effect of different salinity concentrations on content of Chl a (A), Chl b (B), and total Chl (C), as well as on Chl a / b ratio (D), total Car (E), and Chl / Car ratio (F) in trehalose-treated (+Tre) or untreated (-Tre) basil seedlings. Results are the mean ± standard deviation of three separate measurements. Different letters indicate significant differences between the various treatments at P < 0.05 according to the Duncan test.
Although the 25 mM concentration of NaCl had no effect on the amount of Chl b and total Car compared to the control (Fig. 1B, D), it caused a significant increase in the content of Chl a and total Chl by 41 and 30%, respectively (Fig. 1A, C). A significant or non-significant increase in response to 25 mM salinity was observed in Chl a/b and Chl/Car ratios, respectively, which decreased or remained unchanged at higher salinities compared to the control (Fig. 1D, E). Tre treatment resulted in a significant decrease in pigments amount and Chl a/b ratio at most salt levels compared to untreated controls (Fig. 1A-E). However, the Chl/Car ratio increased in salt treatments with a NaCl concentration above 25 mM (Fig. 1F).
Effect of salinity and Tre on soluble sugars and starch contents
All salt treatments caused a sharp increase in the content of soluble sugars, including reducing, non-reducing and total sugars, compared to the control (Fig. 2A-C), so that total soluble sugars reached 145.81 mg g-1 FW under 150 mM NaCl (Fig. 2C).
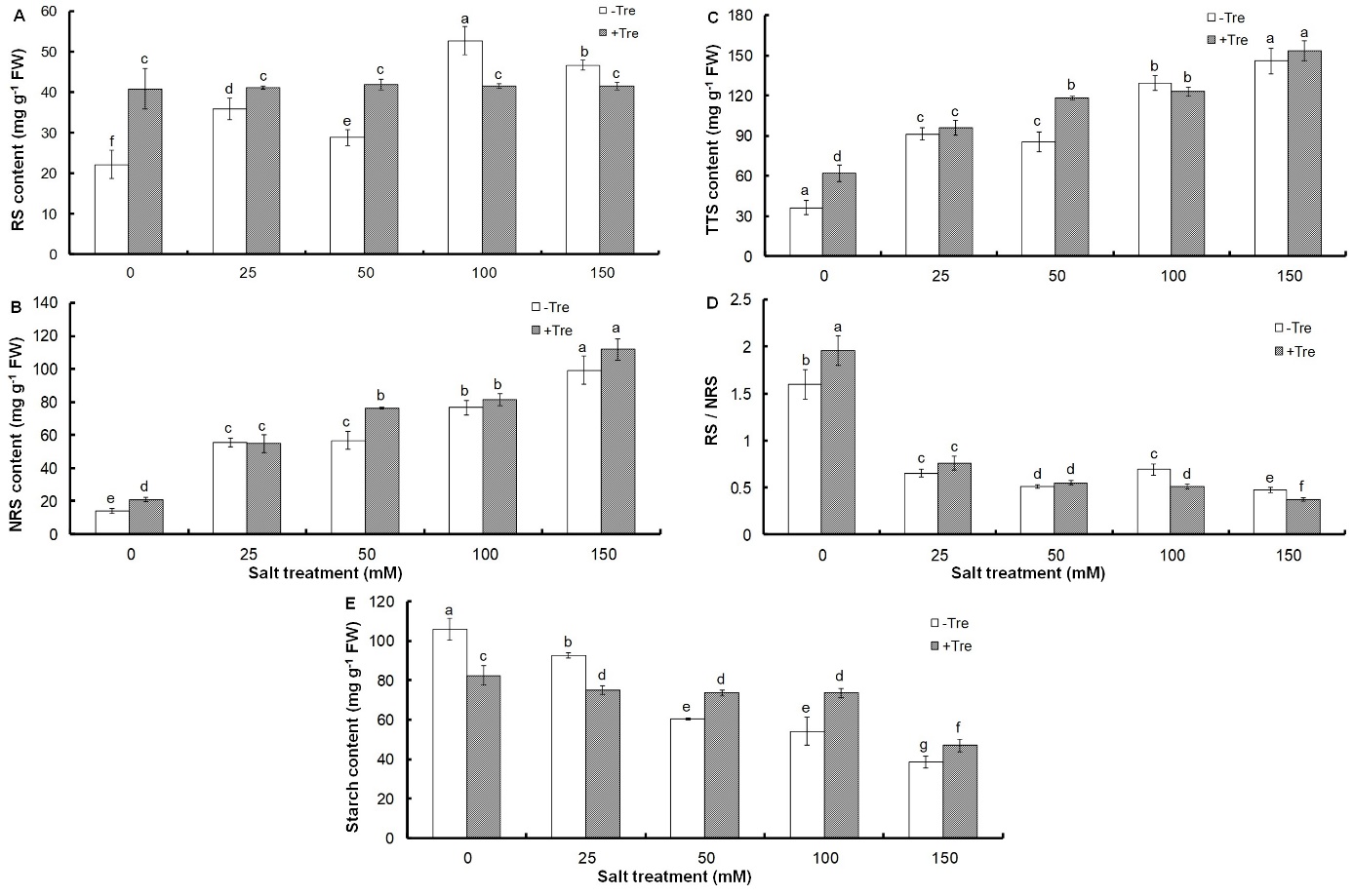
Fig. 2. Effect of different salinity concentrations on content of reducing sugars, RS (A), non-reducing sugars, NRS (B), and total soluble sugars, TSS (C), as well as on reducing / non-reducing sugars, RS/NRS ratio (D), and starch content (E) in trehalose-treated (+Tre) or untreated (-Tre) basil seedlings. Results are the mean ± standard deviation of three separate measurements. Different letters indicate significant differences between the various treatments at P < 0.05 according to the Duncan test.
However, a significant decrease in RS/NRS ratio and starch content was found under all salinities compared to control (Fig. 2D, E). Tre treatment increased soluble sugars in non-stress or salinity conditions up to 50 mM NaCl concentration compared to untreated plants, but at higher salt concentrations, it caused a slight increase in NRS and a significant decrease in RS (Fig. 2A-C). Tre alone increased the ratio of RS/NRS by 22% compared to the untreated control, but did not change this ratio at mild salinity (25 and 50 mM NaCl) or even decreased it to the same extent at 100 and 150 mM NaCl (Fig. 2D). These changes were associated with the accumulation of starch in Tre-treated seedlings at salt concentrations of 50 mM and higher (Fig. 2E).
Effect of salinity and Tre on total proteins, total amino acid, and proline contents
The positive effect of salinity on total protein content was observed only at 25 mM salt concentration with a 75% increase and no significant change was found in other salt treatments, while all salinity levels significantly increased soluble proteins, especially up to 10.88 mg g-1 FW under 50 mM NaCl (Fig. 3A, B).
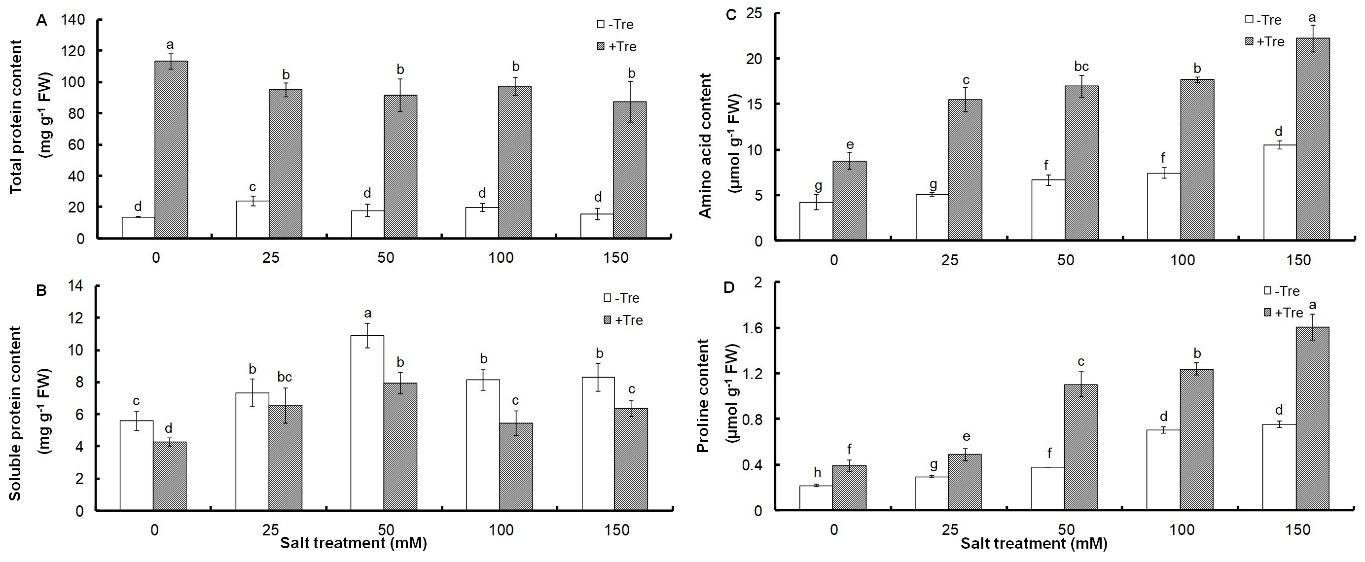
Fig. 3. Effect of different salinity concentrations on contents of total proteins (A), total soluble proteins (B), total amino acids (C), and proline (D) in trehalose-treated (+Tre) or untreated (-Tre) basil seedlings. Results are the mean ± standard deviation of three separate measurements. Different letters indicate significant differences between the various treatments at P < 0.05 according to the Duncan test.
Salinity also caused the accumulation of amino acids and proline so that this increase was proportional to the salt concentration and reached 151 and 247% of control for amino acids and proline in 150 mM NaCl concentration, respectively (Fig. 3C, D). Tre treatment at all salinity concentrations caused a strong increase in total proteins, amino acids, and proline contents, which was associated with a decrease in the concentration of soluble proteins (Fig. 3).
Effect of salinity and Tre on enzyme activities
The activity of antioxidant enzymes including APX, PPX, and PPO increased strongly with salt concentration and reached 144, 179, and 218% at 150 mM NaCl compared to the control (Fig. 4).
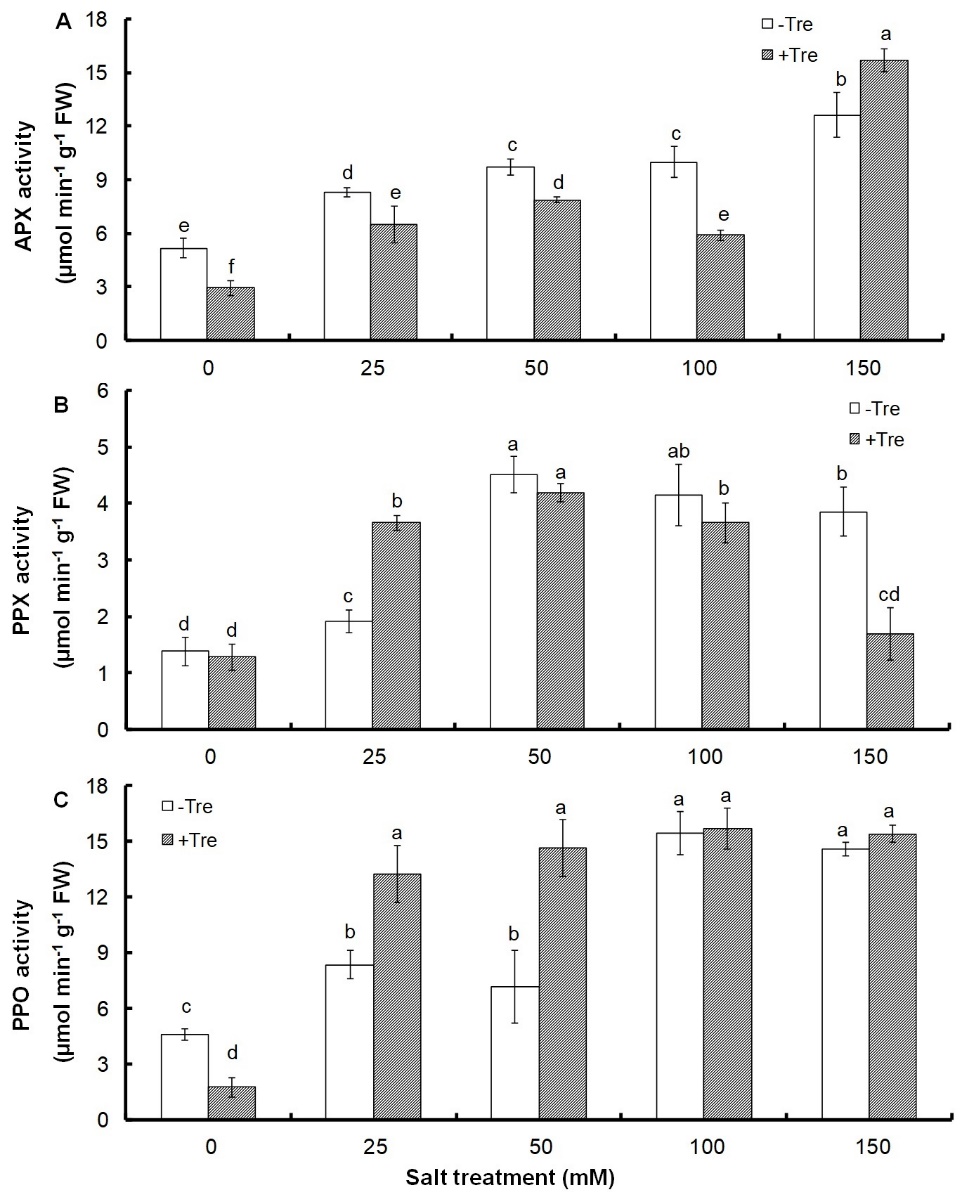
Fig. 4. Effect of different salinity concentrations on the activity of ascorbate peroxidase, APX (A), pyrogallol peroxidase, PPX (B), and polyphenol oxidase, PPO (C) in trehalose-treated (+Tre) or untreated (-Tre) basil seedlings. Results are the mean ± standard deviation of three separate measurements. Different letters indicate significant differences between the various treatments at P < 0.05 according to the Duncan test.
Tre treatment alone or with concentrations of up to 100 mM salt strongly decreased APX activity but increased its activity at 150 mM salt concentration by 24% compared to untreated control (Fig. 4A). PPX enzyme activity did not change in plants treated with Tre alone, but it was strongly increased at 25 mM salinity by 92%, slightly decreased at higher salt concentrations, and significantly decreased at 150 mM salinity by 56% compared to untreated control (Fig. 4B). The activity of PPO enzyme showed a 62% decrease in Tre-treated seedlings compared to untreated ones under non-stress conditions, but it increased significantly by 58 and 104% at concentrations of 25 and 50 mM salt, respectively, and remained unchanged at higher salinities (Fig. 4C).
Discussion
Much research has documented the negative effect of salinity on plant growth and biomass (Ahmad and Jhon 2005, Yoon et al. 2009, Qiu et al. 2014, Ahmad et al. 2018, Scagel et al. 2019, Sheyhakinia et al. 2020). Despite the reduction of some growth indices in basil seedlings under saline conditions, root length, leaf characteristics, and shoot water content did not change and root dry weight increased. Considering the lack of change in the length of the root and also the decrease in the ratio of fresh weight to dry weight of the root under salinity, which is due to a decrease in fresh weight and increase of dry weight, the increase of root biomass can be caused by the accumulation of nutrients in the organ during stress. In fact, salinity causes a change in the pattern of carbon allocation between roots and shoots. This change in the pattern of carbon distribution during salinity is associated with the reduction of shoot biomass. An increase in root biomass and a decrease in shoot biomass under salt stress have been reported (Bernstein and Kafkafi 2002, Imada et al. 2015). Since this process is similar to the responses of roots and shoots to soil water availability (Zhang et al. 2005, McCarthy and Enquist 2007, Imada et al. 2008), one of the reasons is probably the reduction of soil moisture absorption (McCarthy and Enquist 2007). In fact, the inhibition of growth induced by salinity may be related to the reduction of water absorption due to the reduction of soil osmotic potential. Such a phenomenon leads to cell dehydration and loss of turgor pressure, which leads to growth arrest (Zhao et al. 2021). In addition, salinity leads to the accumulation of Na+ and Cl- ions, which reduces the absorption and transport of nutrients due to competitive interactions with their transporters (Zhao et al. 2021, Gao et al. 2022). However, in our results, salinity-induced growth reduction was observed without a change in shoot water content, indicating that this reduction is associated with continuous water uptake and maintenance of shoot turgor pressure, as previously documented (De Costa et al. 2007, Alisofi et al. 2020, Sheyhakinia et al. 2020). Accumulation of proline in basil seedlings under salinity can explain the uninterrupted water uptake and thus the maintenance of shoot water content despite growth limitation. Therefore, changes in carbon partitioning and accumulation of osmolytes to continue water absorption by plants can be the main reason for growth inhibition under saline conditions. The results of studies on Hibiscus sabdariffa (Sheyhakinia et al. 2020) and Momordica charantia (Alisofi et al. 2020) under salt stress are in good agreement with our findings.
Contrary to previous studies on the effect of Tre on plant growth under salinity stress (Mostofa et al. 2015, Abdallah et al. 2020), Tre treatment in most salinities decreased shoot length and fresh and dry weight of shoots and roots in basil seedlings, so that the dry weight reduction was more severe. Early experiments on the effect of Tre on higher plants through inhibition of trehalase (a Tre-degrading enzyme) in species with very low trehalase activity showed that Tre accumulation is toxic or at least Tre acts as a plant growth inhibitor possibly through inhibition of cell wall biosynthesis (Veluthambi et al. 1981, Veluthambi et al. 1982a). This effect is associated with a disturbance in carbohydrate metabolism as indicated by a decrease in sucrose content (Veluthambi et al. 1982b). Therefore, the reduction of growth in basil seedlings due to Tre treatment can be related to the low activity of this enzyme. Meanwhile, in plant species with high trehalase enzyme activity, such as Raphanus sativus, Quamoclit phoenicea, and Zea mays, toxicity and inhibition of growth by Tre were not observed (Veluthambi et al. 1981). In transgenic Arabidopsis seedlings in which trehalase enzyme activity was overexpressed, there was no effect of growth inhibition in the presence of Tre, unlike the control (Schluepmann et al. 2004). Therefore, the decrease of growth induced by Tre can be due to the plant's inability to metabolize it, which leads to the accumulation of trehalose-6-phosphate and subsequently to the reduction of the glucose-6-phosphate pool (Schluepmann et al. al. 2004). In addition, it has been found that trehalose-6-phosphate induces plant defense responses, which are associated with growth reduction (Reignault et al. 2001, Brodmann et al. 2002, Renard-Merlier et al. 2007). However, Tre did not affect SWC in basil seedlings up to 100 mM NaCl, but increased it in seedlings exposed to 150 mM NaCl. This event is associated with proline hyperaccumulation, so it can be concluded that changes in carbon partitioning and directing it to proline biosynthesis are the reason for Tre-induced growth inhibition under saline conditions. Considering the role of Tre and its metabolism in the regulation of growth and development (Nunes et al. 2013, O'Hara et al. 2013), it is likely that this metabolite is involved in the growth regulation processes of basil seedlings. In this regard, it has recently been found that methyl jasmonate treatment not only does not reduce the harmful effects of salinity stress in radish ( Raphanus sativus) but can even inhibit plant growth (Henschel et al. 2023). This shows that signal molecules and osmolytes such as Tre, which usually increase tolerance to environmental stresses, do not work in the same way in all plants and their performance against different stresses can depend on the type of plant species.
It has been found that the concentration of photosynthetic pigments is sensitive to environmental stresses and in most plants, it decreases significantly in response to salinity (Ahmad and Jhon 2005, Gunes et al. 2007, Yoon et al. 2009, Qiu et al. 2014). However, a study on tomato has shown that 0.3 M salt concentration induces Chl production per unit of leaf area (Agong et al. 2004). This shows that very low salinity induces Chl biosynthesis in basil, as observed in the concentration of Chl a and total Chl at 25 mM NaCl. In addition, the observed decrease in Chl a/b and Chl / Car ratios in response to salinity shows the greater sensitivity of Chl a compare to Chl b and Chl to Car. Contrary to current studies on the role of Tre in increasing the photosynthetic pigments of different plants under salinity (Theerakulpisut and Phongngarm 2013, Abdallah et al. 2016, 2020), treatment of basil seedlings with Tre in most salinities showed a significant decrease in pigment content compared to untreated plants. The increase in the ratio of Chl a/b in Tre-treated seedlings under non-stress conditions or not changing it at the concentration of 25 mM indicates the greater sensitivity of Chl b to Tre. It has been found that the biosynthesis of Chl b is carried out through the oxidation of a methyl group to a formyl group on the B ring of the Chl a molecule (Porra et al. 1994). Therefore, it can be assumed that the enzyme(s) involved in Chl a biosynthesis are more active than Chl b due to Tre treatment alone or in combination with low salinity. However, the sharp decrease of this ratio in salt concentrations of 50 mM and higher shows the greater sensitivity of Chl a to Tre. The change pattern of the Chl to Car ratio also shows the lower sensitivity of Car to Tre in non-stressed conditions or in combination with low salinity and its greater sensitivity in higher salinity concentrations. Considering the negative effect of Tre on the growth of basil seedlings under salt stress, the reduction of photosynthetic pigments can be attributed to the inability of plants to properly metabolize Tre or direct carbon to other metabolic pathways.
Accumulation of sugars or osmotic regulators in plant cells under salinity is a strategy to control plant water content and inhibit water loss (Chaves et al. 2009). The role of the accumulation of soluble sugars in salinity stress tolerance has been widely studied (Mishra et al. 2008, Yin et al. 2010). This indicates the type of basil strategy in salinity tolerance that is done through the accumulation of soluble sugars. Considering the sharp decrease in the ratio of RS/NRS under salt stress compared to the control, it can be concluded that the accumulation of NRS occurs at a higher rate than RS during salt treatment. In addition, the numerical comparison of the amount of these sugars in response to different salt concentrations shows that the accumulation of NRS is almost twice as much as RS. This indicates the important role of non-reducing sugars in basil seedlings for salinity tolerance. On the other hand, the increase of soluble sugars during salinity stress is associated with the decrease in starch content. This suggests that a change in photosynthetic carbon partitioning occurs in basil seedlings during salinity stress, leading to more sucrose synthesis and less starch accumulation. The higher accumulation of NRS than RS confirms this. However, starch breakdown can also increase soluble sugars during salt stress. The conversion of starch to sugars, especially NRS, and a change in their metabolism, which has been introduced as a common defense strategy against water stress, is possible through increasing the activity of starch hydrolyzing enzymes such as amylases and simultaneous decrease of sucrose hydrolyzing activities (Kumari and Asthir 2016). The unremarkable increase of NRS content in Tre-treated seedlings under salinity compared to the untreated controls indicates that this metabolite does not accumulate in basil seedlings. This finding can confirm the hypothesis that Tre accumulates in some Tre-treated plants in another form such as trehalose-6-phosphate (Schluepmann et al. 2004). This proves that the effects of Tre on growth are due to changes in carbohydrate metabolism. Evidence to support this hypothesis comes from the observation that the simultaneous addition of sucrose in the presence of Tre and starch accumulation restores growth in response to Tre feeding (Wingler et al. 2000). Tre feeding leads to an increase in ADP-glucose pyrophosphorylase gene expression as well as an increase in its enzyme activity, which is crucial in starch biosynthesis (Wingler et al. 2000). In addition, trehalose-6-phosphate also plays a role in the activation of this enzyme (Kolbe et al. 2005). Various studies have shown that growth was impaired after an increase of the gene expression of this enzyme in potato plants, but with the addition of sucrose, growth returned to the normal state (Stark et al. 1992). Accordingly, one of the reasons for Tre-induced growth inhibition during salt treatment can be due to the reduction of carbon for export to growth areas because of starch accumulation.
Environmental stresses, including salinity, generally cause protein degradation or reduced synthesis due to the acceleration of the aging process (Mishra et al. 2008, Misra and Saxena 2009). The results of our study on basil seedlings showed the opposite of this finding, which could indicate an increase in the synthesis of stress-specific proteins or enzyme proteins during the salinity period. The observed decrease in soluble protein levels, which was accompanied by a significant increase in total protein content in Tre-treated seedlings, could be attributed to a change in their solubility due to Tre treatment. This suggestion is supported by the fact that the level of soluble protein is positively correlated with protein solubility (Afify et al. 2012, Ma et al. 2019, Ebert et al. 2020). Therefore, the increase in total protein while the soluble protein decreased can be due to the role of Tre in reducing the solubility of proteins. The response of basil seedlings to Tre treatment can confirm that the pathway of carbon partitioning and its metabolism is changed due to Tre treatment, which in turn reduces growth in Tre-treated seedlings under salinity conditions. Similar results regarding the effects of Tre on salinity tolerance and its role in carbon partitioning have also been reported in Dunaliella bardawil (Panjekobi and Einali 2021).
Accumulation of amino acids and proline is considered a common response during environmental stress, and is often associated with the improvement of plant tolerance to stress conditions (Claussen 2005, Khadri et al. 2006, Yoon et al. 2009). However, some studies have shown that the level of accumulated proline indicates the severity of stress symptoms when plants are exposed to different types of abiotic stresses (Metwally et al. 2003, Mostofa et al. 2014, 2015). A study on Cathranthus roseus under salt stress showed that proline accumulation under stress conditions is negatively correlated with relative water content, biomass, and potassium accumulation (Chang et al. 2014). However, the lack of change in SWC in saline conditions or its increase due to Tre treatment and high salt dose, which is associated with proline accumulation, can indicate the increase of this metabolite in order to maintain the water content of the plant. Therefore, in our study, the excessive accumulation of amino acids and proline that occurred especially due to Tre treatment is related to the maintenance of water content, which is caused by Tre-induced metabolic changes during salt stress. In this way, the increase of these metabolites in seedlings treated with Tre alone also indicates a kind of stressful condition. On the contrary, studies on plants treated with Tre under salt (Nounjan et al. 2012, Mostofa et al. 2014, Sadak, 2019) and drought stress (Ali and Ashraf 2011) show a lower level of proline or amino acid in these plants, which could indicate a lower demand for proline or a compensatory mechanism for Tre, because both can act as an osmoprotectant.
The increased activity of antioxidant enzymes in response to salinity can be considered as an indication of increased ROS production and a common protective mechanism to reduce oxidative damage caused by salinity. These results are consistent with other studies on the role of ROS-scavenging enzymes under salt stress (Ahmad et al. 2018, Alisofi et al. 2020, Sheyhakinia et al. 2020). Different activities of antioxidant enzymes in response to Tre under salt stress have been reported in various plants. Tre treatment decreased the activity of peroxidase, catalase, and superoxide dismutase enzymes in rice under salt stress (Rohman et al. 2019). In another study, the treatment of Chenopodium quinoa with Tre under salt stress increased the activity of APX, catalase and superoxide dismutase enzymes (Abdallah et al. 2020). In addition, an increase in the activity of antioxidant enzymes has been observed with Tre treatment under different stress conditions (Zhao et al. 2019, Liu et al. 2020). However, in all these studies, Tre-treated plants showed tolerance to salt stress, which can indicate the existence of different strategies in plants to cope with salinity in the presence of Tre. In contrast, our results showed that the changes in enzyme activity patterns induced by Tre treatment do not indicate increased tolerance to salt stress, because these patterns are not correlated with other indicators showing stress reduction.
Conclusion
The results of this study show that salt stress without change in SWC decreases growth characteristics, photosynthetic pigments, and starch content, but increases the activity of ROS-scavenging enzymes and the accumulation of metabolites including soluble sugars, proteins, free amino acids, and proline. Tre treatment not only does not reduce the adverse effects of salinity but even causes more severe inhibition of plant growth, further reduction of photosynthetic pigments and soluble proteins along with the excessive accumulation of free amino acids and proline. Therefore, Tre treatment is not effective in salinity tolerance of basil seedlings and reduces their growth possibly through diverting carbon to other metabolic pathways rather than growth processes. However, due to the effect of Tre on the change in carbon partitioning, the role of this molecule in various metabolic and physiological pathways is obvious, the detailed understanding of which requires further research on the physiological effects of Tre under stress and non-stress conditions.
Acknowledgements
We thank the Deputy of Research at the University of Sistan and Baluchestan for financial support in the form of grants for the MSc research project.